-
Theme 1: At the beginning
Genomic imprinting is a mammalian-specific epigenetic mechanism regulating the parent-of-origin-specific expression of a subset of genes. It was independently discovered in 1984...
-
Theme 2: Imprinted genes, PEGs and MEGs
We returned to Japan with materials provided by Azim Surani, such as parthenogenetic embryos and control, normally fertilized embryos...
-
Theme 3: The origin of DMR
The imprinted regions are comprised of clusters of imprinted genes, meaning that the imprinted genes are regulated at the regional level, not in a gene-by-gene manner. Each imprinted region...
-
Theme 4: PEG10, a therian-specific exapted gene, plays an essential role in placenta formation
We assumed that the imprinted gene essential for placenta formation would exist in the imprinted region on mouse proximal chromosome 6 because this is the only imprinted region...
-
Theme 5: PEG11/RTL1 and SIRH/RTL are eutherian-specific exapted genes, and their functions are related to eutherian-specific traits
Maternal duplication of the distal chromosome 12 causes late stage embryonic/ neonatal lethality...
-
Theme 6: Biological evolution in mammals: perspectives on genomic imprinting as well as mammalian-specific genes exapted from LTR retrotransposon/retrovirus
Why has genomic imprinting been so widely conserved in mammals? Genomic imprinting is seemingly contradictory...
-
Theme 7: PEG11/RTL1 contributions to muscle development and brain functions in the course of eutherian evolution.
In Theme 5, we showed that Peg11/Rtl1 plays an essential role in the maintenance of placental...
-
Theme 8: Genes expressed in neurons that regulate brain function— Sirh4, 5, 6/Rtl8c, a, b—
Among the SIRH/RTL genes functioning in neurons, PEG11/RTL1is known as the causative gene for Kagami-Ogata syndrome and Temple syndrome...
-
Theme 9: Genes expressed in microglia, the immune cells of the brain—Sirh3, Sirh8, Sirh10 and Sirh11—
Innate immunity is a fundamental biological defense system, widely conserved across the animal kingdom...
-
Theme 10: The placenta as a laboratory for evolution—The role of the placenta in further acquisition of virus-derived genes—
“The placenta”serves as a fascinating example of an innovative evolution, where virus-derived genes have been...
Theme 1: At the beginning
-An approach to the biological meaning of genomic imprinting based on a systematic screening of imprinted genes-
Genomic imprinting is a mammalian-specific epigenetic mechanism regulating the parent-of-origin-specific expression of a subset of genes. It was independently discovered in 1984 by the Surani, Solter and Lovell-Badge groups, demonstrating that mammals never develop to term without both of the parental genomes: embryos with either two maternally or paternally derived genomes, such as the case with parthenogenetic or androgenetic embryos, exhibit early embryonic lethality (Fig. 1)1-3. A series of genetic studies by Cattanach’s group using mice with Robertsonian translocations also provided strong evidence for functional differences between the parental chromosomes: mice with uniparental duplication of certain specific chromosomal regions (the “imprinted regions”) displayed a variety of defects in development, growth and/or behavior (Fig. 2)4,5. Approximately 20 such chromosomal imprinted regions have been identified in mice.
We, my collaborator Fumitoshi Ishino and I, had the idea that the functional differences between the paternally and maternally derived genomes are due to the presence of genes exhibiting parent-of-origin-specific monoallelic expression (now called “imprinted genes”). We started our genomic imprinting research in Azim Surani’s laboratory in Cambridge in 1990 for the identification of such imprinted genes by developing a novel systematic screening method. In order to elucidate the biological meaning of genomic imprinting, we knew it would be critically important to determine the common biological function(s) of the imprinted genes.
At that time, we expected that at least four categories of imprinted genes would be identified:
- Genes derived from exogenous DNA, because I assumed that genomic imprinting arose as a genome defense mechanism against the integration of exogenous DNA, such as viral DNA, into the mammalian genome.
- Genes specific to mammals, because genomic imprinting is a mammalian-specific mechanism.
- Genes involved in the formation and function of the placenta, because both parthenogenetic and androgenetic embryos exhibit severe but also different abnormalities in the placenta.
- Genes involved in immunological tolerance in pregnancy, because both embryos and placentas are semiallogenic to the mother.
I myself expected that a considerable number of imprinted genes in category 1 would be identified by our screening efforts.
This is the concept behind our research.
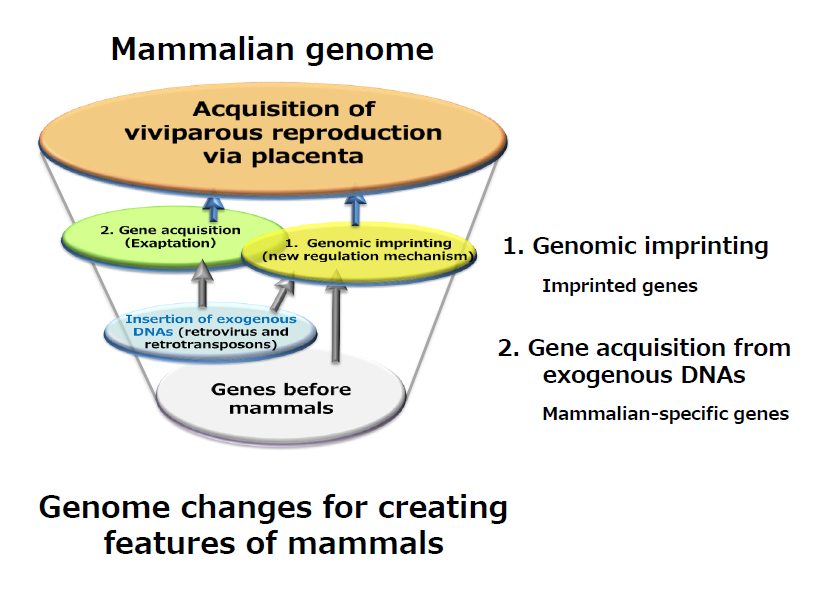
We first sought to identify genes that satisfied two conditions: one that it is an imprinted, newly regulated gene (the yellow oval), and that is an exapted, newly acquired gene in mammals (the light green oval). This is because we assume that such genes must have contributed to the establishment of representative mammalian features of mammals, such as viviparity via the emergence of placenta.
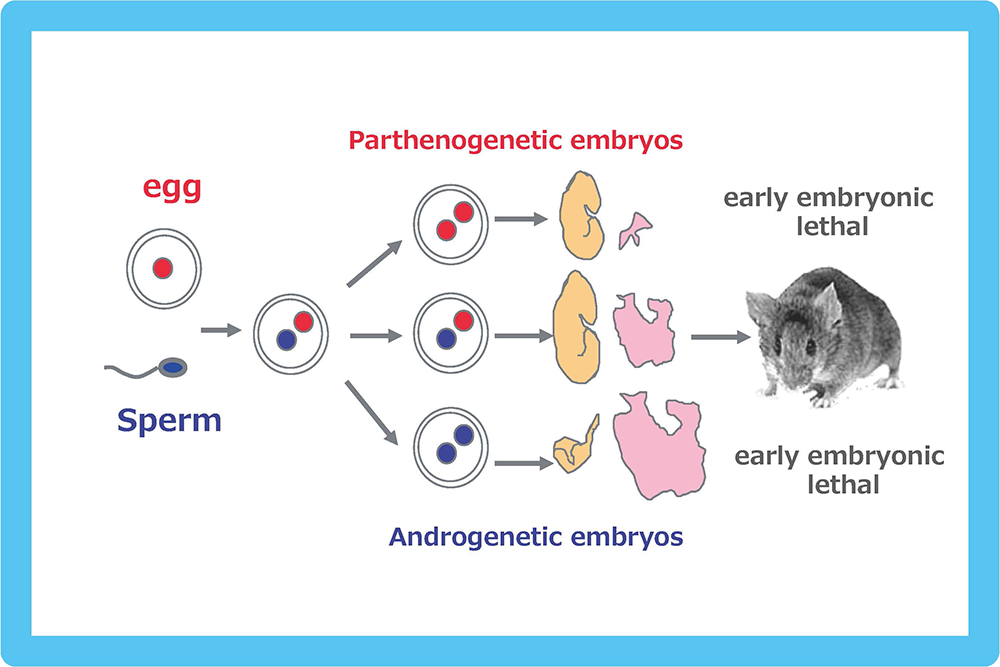
Both the parthenogenetic and androgenetic embryos produced by pronuclear transplantation experiments exhibit early embryonic lethality with totally different morphological defects on developmental day 10.5. In both cases, placental development was severely disturbed.
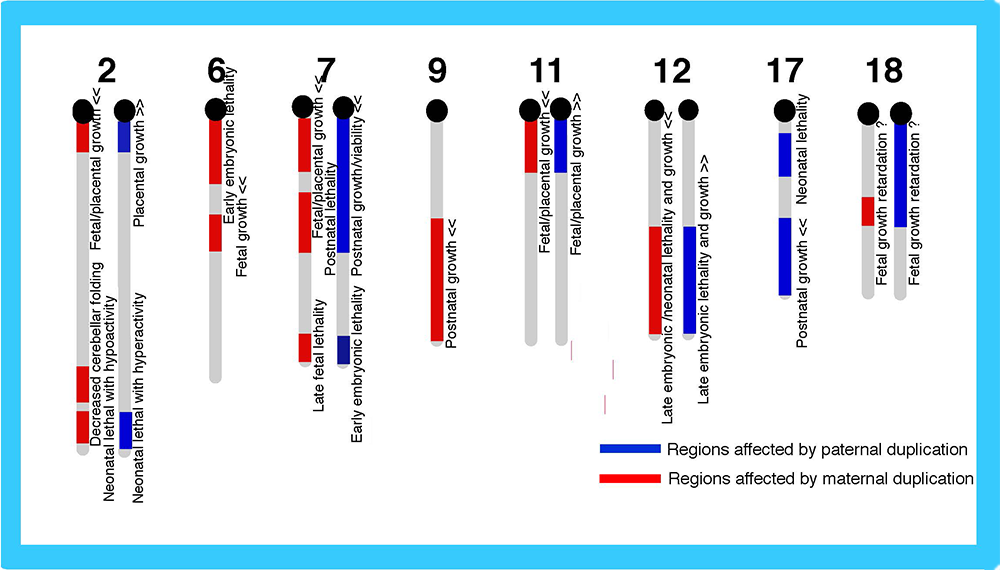
A mouse genomic imprinting map deduced from a series of Robertosonian translocation experiments (called imprinted regions). A variety of the abnormal phenotypes observed in mice with partial uniparental duplications are shown, such as lethality in several different developmental stages, as well as growth and behavioral abnormalities. Modified from data in ref. 5.
References
- Surani M A, Barton S C and Norris M L. (1984) Development of reconstituted mouse eggs suggests imprinting of the genome during gametogenesis. Nature 308, 548-550.
- McGrath J and Soter D. (1984) Completion of mouse embryogenesis requires both the maternal and paternal genomes. Cell 37, 179-183.
- Mann J R and Lovell-Badge R H. (1984) Inviability of parthenogenones is determined by pronuclei, not egg cytoplasm. Nature 310, 66-67.
- Cattanach B M and Kirk M. (1985) Differential activity of maternally and paternally derived chromosome regions in mice. Nature 315, 496–498.
- Cattanach B M and Beechey C V. (1990) Autosomal and X-chromosome imprinting. Development Suppl, 63-72.
Theme 2: Imprinted genes, PEGs and MEGs
We returned to Japan with materials provided by Azim Surani, such as parthenogenetic embryos and control, normally fertilized embryos (Fig. 1). We then developed a new method and conducted a systematic screening for paternally expressed genes (PEGs) by subtracting the genes expressed in parthenogenetic embryos from those in normally fertilized embryos6. Using essentially this method, we identified 12 mouse Pegs and mapped them to 8 imprinted regions6-13 (Fig. 3). We also conducted another systematic screening for maternally expressed genes (MEGs) and identified 11 mouse Megs in 5 imprinted regions13-15 (Fig. 3). These results demonstrated that the genomic imprinting phenomena discovered by developmental engineering (Fig. 1) and genetic experiments (Fig. 2) have the same basis, thereby contributing to the establishment of the current concept that genomic imprinting is attributable to the existence of PEGs and MEGs (Fig. 3). A number of imprinted genes were also reported by other groups and it turned out that imprinted regions are gene clusters comprising both PEGs and MEGs.
At first, it seemed that imprinted genes fit well with “the conflict hypothesis” proposed by Haig’s group16 that states that paternally expressed genes promote embryonic growth, while maternally expressed genes inhibit embryonic growth as a consequence of the genetic conflict between the paternal and maternal alleles during mammalian evolution. In support of this concept, the first two imprinted genes, Igf2r (MEG) and Igf2 (PEG) are very good examples. However, as the imprinted genes increased in number, exceptions also increased, because many of their knockout (KO) mice models did not exhibit any evident growth abnormalities nor distinct phenotype. Even so, a substantial number of imprinted genes did fit with this prediction, so this concept has come to be widely accepted. It also became difficult to conclude the common biological or biochemical functions among the imprinted genes. However, by analyzing their expression profiles, we found that all the imprinted genes exhibit placental expression even when their placental functions were unknown, and so we proposed “a novel placenta hypothesis”17.
We identified Peg1/Mest6 and Peg37 in our first screening. Their KO embryos and neonates displayed a growth retardation phenotype18,19, that is, they are also good examples of the conflict hypothesis. Additionally, their female KO mice displayed an interesting phenotype, i.e. a lack of the normal maternal behavior of taking care of their pups, when the KO allele was transmitted paternally18,19, suggesting they also function in the brain. Contrary to my personal expectation, we were able to identify only two imprinted genes derived from exogenous DNA, PEG10 and PEG11/RTL1.
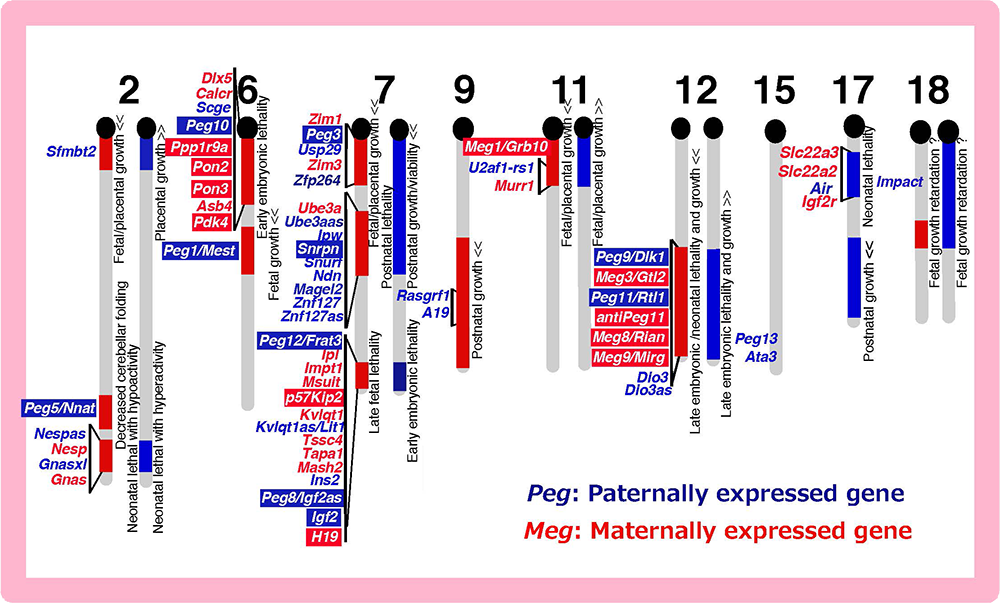
The imprinted regions comprise both Pegs (blue) and Megs (red). Peg10 was mapped in the imprinted region on proximal chromosome 6 associated with early embryonic lethality upon maternal duplication, and Peg11/Rtl1 in the region on distal chromosome 12 associated with late embryonic/neonatal lethality upon maternal duplication and late embryonic lethality upon paternal duplication.
References
- Kaneko-Ishino T, Kuroiwa Y, Miyoshi N, Kohda T, Suzuki R, Yokoyama M, Viville S, Barton S C, Ishino F* and Surani A*. Peg1/Mest imprinted gene on Chromosome 6 identified by cDNA subtraction hybridization. Nat Genet 11, 52-59 (1995).
- Kuroiwa Y, Kaneko-Ishino T, Kagitani F, Kohda T, Li L-L, Tada M, Suzuki R, Yokoyama M, Shiroishi T, Wakana S, Barton S C, Ishino F* and Surani A*. Peg3 imprinted gene on proximal chromosome 7, encodes for a zinc finger protein. Nat Genet 12, 186-190 (1996).
- Kagitani F, Kuroiwa Y, Wakana S, Shiroishi T, Kobayashi S, Kohda T, Kaneko-Ishino T and Ishino F. Peg5/Neuronatin is an imprinted gene located on sub-distal chromosome 2 in the mouse. Nucl Acids Res 25, 3428-3432 (1997).
- Okutsu T, Kuroiwa Y, Kagitani F, Kai M, Aisaka K, Tsutsumi O, Kaneko Y, Yokomori K, Surani M A, Kohda T, Kaneko-Ishino T and Ishino F. Expression and imprinting status of human PEG8/IGF2AS, a paternally expressed antisense transcript from the IGF2 locus, in Wilms' tumors. J Biochemistry 127, 475-483 (2000).
- Kobayashi S, Wagatsuma H, Ono R, Ichikawa H, Yamazaki M, Tashiro H, Aisaka K, Miyoshi N, Kohda T, Ogura A, Ohki M, Kaneko-Ishino T and Ishino F. Mouse Peg9/Dlk1 and human PEG9/DLK1 are paternally expressed imprinted genes closely located to the maternally expressed imprinted genes: mouse Meg3/Gtl2 and human MEG3. Genes to Cells 5, 1029-1037 (2000).
- Ono R, Kobayashi S, Wagatsuma H, Aisaka K, Kohda T, Kaneko-Ishino T and Ishino F. A retrotransposon-derived gene, PEG10, is a novel imprinted gene located on human chromosome 7q21. Genomics 73, 232-237 (2001).
- Kobayashi S, Kohda T, Ichikawa H, Ogura A, Ohki M, Kaneko-Ishino T and Ishino F. Paternal expression of a novel imprinted gene, Peg12/Frat3, in the mouse 7C region homologous to the Prader-Willi syndrome region. Biochem Biophys Res Commun 290, 403-408 (2002).
- Ono R, Shiura H, Abratani Y, Kohda T, Kaneko-Ishino T and Ishino F. Identification of a large novel imprinted gene cluster on mouse proximal chromosome 6. Genome Res 13, 1696-1705 (2003).
- Miyoshi N, Kuroiwa Y, Kohda T, Shitara H, Yonekawa H, Kawabe T, Hasegawa H, Barton S C, Surani M A, Kaneko-Ishino T and Ishino F. Identification of the Meg1/Grb10 imprinted gene on mouse proximal chromosome 11, a candidate for the Silver-Russell syndrome gene. Proc Natl Acad Sci USA 95, 1102-1107 (1998).
- Miyoshi N, Wagatsuma H, Wakana S, Shiroishi T, Nomura M, Aisaka K, Kohda T, Surani M A, Kaneko-Ishino T and Ishino F. Identification of an imprinted gene, Meg3/Gtl2 and its human homologue MEG3, first mapped on mouse distal chromosome 12 and human chromosome 14q. Genes to Cells 5, 211-220 (2000).
- Moore T and Haig D. Genomic imprinting in mammalian development: a parental tug-of-war. Trends Genet 7, 45-49 (1991).
- Kaneko-Ishino T, Kohda T and Ishino F. The regulation and biological significance of genomic imprinting in mammals. J Biochem 133, 699-711 (2003).
- Lefebvre L, Viville S, Barton S C, Ishino F, Keverne E B and Surani M A. Abnormal maternal behaviour and growth retardation associated with loss of the imprinted gene Mest. Nat Genet 20, 163-169 (1998).
- Li L-L, Keverne E B, Apricio S A, Ishino F, Barton S C and Surani M A. Regulation of maternal behavior and offspring growth by paternally expressed Peg3. Science 284, 330-333 (1999).
Theme 3: The origin of DMR
-The second approach to elucidating the biological meaning of genomic imprinting from a monoallelic expression mechanism-
The imprinted regions are comprised of clusters of imprinted genes, meaning that the imprinted genes are regulated at the regional level, not in a gene-by-gene manner. Each imprinted region has its own differentially methylated region (DMR) that plays a role as an imprinting control region (ICR), regulating the reciprocal expression of PEGs and MEGs from the paternal and maternal chromosomes, respectively17,20,21 (Fig. 4). Thus, the essence of genomic imprinting is that two parental chromosomes having a different methylation status are required for the expression of PEGs and MEGs22,23 to properly support normal development in mammals17.
How was this unique mechanism established in mammals in the course of evolution? In the insulator model22,23 (Fig. 4), the reciprocal regulation mechanism comprises 5 fundamental components, i.e. a promoter, insulator, enhancer, DMR and insulator binding protein, such as CTCF, which bind to the insulator sequence. This means that the reciprocal expression mechanism is intrinsically installed in the mammalian genome because the first four are cis-functional genome units, while the last is ubiquitous in eukaryotes. Among them, the DMR is the only mammalian-specific component, implying it is a key factor in this mechanism. However, no one had elucidated any conserved sequences nor common feature(s) among the DMRs, so this question remained unsolved for a long period of time.
An important clue to resolving this issue came from an analysis of PEG10. PEG10 was derived from a certain LTR retrotransposon or retrovirus (see Theme 4). By a series of collaboration studies with Marilyn Renfree’s group in Australia, we demonstrated that PEG10 is conserved in marsupials as well as eutherians and exhibits paternal expression in both groups24. Upon comparing the PEG10 region in these two groups as well as other vertebrates, we found that in addition to PEG10 itself, the PEG10-DMR in its promoter regionis also comprised of a sequence newly inserted into the therian genome, as shown by the CpG profile in the area around this region24 (Fig. 5). Importantly, the insertion of the PEG10-DMR sequence closely corresponds to the emergence of PEG10 imprinting regulation in therians24. Using essentially the same analyses, we eventually came to the conclusion that the DMRs were actually derived from inserted sequences and that each insertion event occurred at the time when the imprinting regulation started25-28 (Fig. 6). For example, most of the DMRs in the eutherian-specific imprinted regions emerged in the eutherian genome. Two exceptions are the Slc38a4- and Snrpn-DMRs because their sequences already existed before the imprinting regulation started, that is, before the diversification of the therians and monotremes (for the former), and before diversification of the eutherians and marsupials (for the latter) (Fig. 6). What happened in these two cases? Interestingly, chromosomal rearrangements occurred close to these DMRs in eutherians25,29. Thus, the origin of the DMRs is associated with large, accidental structural changes in chromosomes, such as the insertion of new DNA sequences or chromosome rearrangements.
The DMRs are known to have certain cis-regulatory functions, such as their activity as promoters, insulators and silencers, and thus are able to interfere with normal gene expression around the insertion sites. DNA methylation of the DMRs normalizes this unfavorable situation to a certain extent, although concomitant extra-repression of surrounding genes may be associated with their presence. This indicates that the genomic imprinting mechanism is a defense mechanism of the genome against the insertion of such foreign DNA sequences having potent cis-acting function(s). We first expected that the insertion of exogenous DNAs would result in the emergence of new imprinted genes (Theme 1), but it turns out to be highly likely that it was the insertion of exogenous DNA with cis-acting functions that resulted in the emergence of new imprinted regions, with their cis-acting parts serving as the DMRs. The genome defense hypothesis is thus revived with a necessary modification.
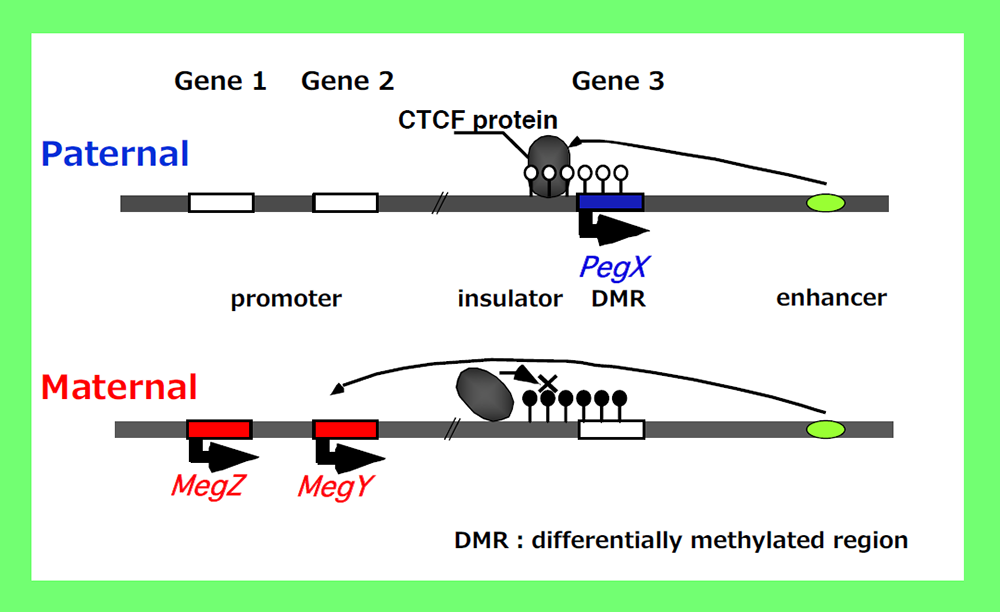
According to “the insulator model” proposed by Bell and Felsenfeld22 as well as Tilghman’s group23, Peg-X is expressed only from the non-methylated paternal chromosome while MegY and MegZ are repressed because insulator binding proteins, such as CTCF, bind to the DMR and block the activity of a downstream enhancer. In contrast, the expression of MegY and MegZ is induced from the methylated maternal chromosome because the insulator binding proteins cannot bind to the methylated DMR, which simultaneously inhibits Peg-X expression because of promoter methylation. The reciprocal regulation of PEGs and MEGs by DMR methylation of one of the parental chromosomes (the maternal chromosome in this case) means that PEGs and MEGs cannot be expressed from a single chromosome. Therefore, two different epigenomes (of the paternal and maternal chromosomes with or without DNA methylation of the DMR) is required for the expression of PEGs and MEGs17.
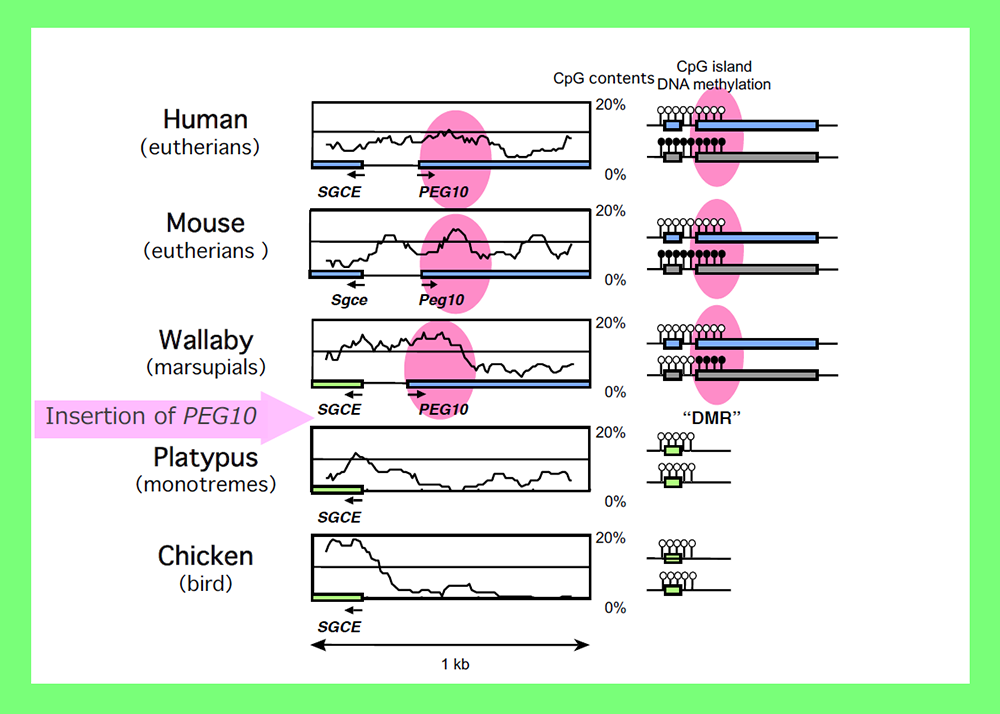
The CpG profiles around the PEG10 promoter demonstrated that this part was derived from a newly inserted DNA and corresponds to the PEG10-DMR region (shown as pink circles). Modified from Fig. 5 in ref. 24.
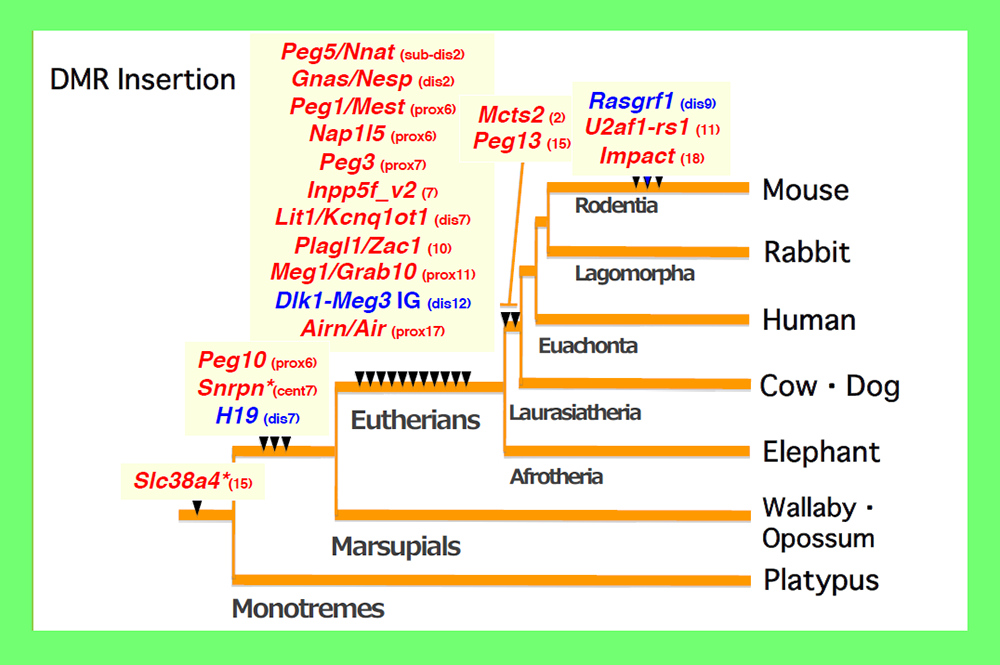
Paternally and maternally methylated DMRs are shown in blue and red, respectively. The locations of the mouse chromosomes are shown. Only the PEG10 and H19-IGF2 DMRs are common to the eutherians and marsupials. *The imprinted regulation of these two regions started in eutherians associated with chromosome rearrangements near the DMRs. Modified from Fig.6 in ref. 25.
References
- Reik W and Walter J. Genomic imprinting: parental influence on the genome. Nat Rev Genet 2, 21–32 (2001).
- Barlow D P and Bartolomei M S. Genomic imprinting in Mammals. Cold Spring Harb Symp Quant Biol 69, 357-375 (2004).
- Bell A C and Felsenfeld G. Methylation of a CTCF-dependent boundary controls imprinted expression of the Igf2 gene. Nature 405, 482-485 (2000).
- Hark A T, Schoenherr C J, Katz D J, Ingram R S, Levorse J M, Tilghman S M. CTCF mediates methylation-sensitive enhancer-blocking activity at the H19/Igf2 locus. Nature 405, 486-489 (2000).
- Suzuki S, Ono R, Narita T, Pask A J, Shaw G, Wang C, Kohda T, Alsop A E, Graves M J A, Kohara Y, Ishino F*, Renfree M B* and Kaneko-Ishino T*. Retrotransposon silencing by DNA methylation can drive mammalian genomic imprinting. PLoS Genet 3, e55 (2007).
- Suzuki S, Shaw G, Kaneko-Ishino T, Ishino F and Renfree M B. (2011) The evolution of mammalian genomic imprinting was accompanied by the acquisition of novel CpG islands. Genome Biol Evol 3, 1276-1283.
- Renfree M B, Suzuki S and Kaneko-Ishino T. The origin and evolution of genomic imprinting and viviparity in mammals. Philos Trans R Soc Lond Biol Sci 368, 20120151 (2013).
- Kaneko-Ishino T and Ishino F. Mammalian-specific genomic functions: Newly acquired traits generated by genomic imprinting and LTR retrotransposon-derived genes in mammals. Proc Jpn Acad Ser B Phys Biol Sci 91, 511-538 (2015).
- Kaneko-Ishino T and Ishino F. Evolution of viviparaity in mammals: what genomic imprinting tells us about mammalian placental evolution. Reprod Fertil Dev (2019). doi: 10.1071/RD18127.
- Hore T A, Rapkins R W and Graves J A M. Construction and evolution of imprinted loci in mammals. Trends Genet 23, 440-448 (2007).
Theme 4: PEG10, a therian-specific exapted gene, plays an essential role in placenta formation
We assumed that the imprinted gene essential for placenta formation would exist in the imprinted region on mouse proximal chromosome 6 because this is the only imprinted region where maternal duplication causes early embryonic lethality as occurs in parthenogenetic embryos (Fig. 2). We found PEG10 in the orthlogous imprinted region on human 7q2111. Interestingly, PEG10 is a gene derived from a certain LTR retrotransposon or retrovirus because it encodes two open reading frames exhibiting high homology to the Gag and Pol proteins of the sushi-ichi retrotransposon, respectively, and the -1 frameshift mechanism which produces a Gag-Pol fusion protein that is unique to LTR retrotransposons and retroviruses is conserved in PEG1011. We knew that PEG10 is conserved in humans and mice but absent from chicken and fish, suggesting that it is a mammalian-specific gene. We obtained Peg10 KO mice to see whether this LTR retrotransposon/retrotransposon-derived gene really functions as an essential placental gene and confirmed that the KO fetuses exhibit early embryonic lethality due to severe placental defects, i.e. a lack of the labyrinth and spongiotrophoblast layers in the placenta30 (Fig. 7). Thus, it turned out that PEG10 has characteristics that fulfill three out of the four conditions we first assumed, that is, it is an exogenous, mammalian-specific gene that has essential placental function (Theme 1).
We analyzed the PEG10 regions in monotremes and marsupials in collaboration with the Renfree and Graves groups, and demonstrated that PEG10 is conserved in the eutherian and marsupial mammals among the vertebrates (Fig. 8). It exhibits imprinted paternal expression in both groups and its promoter acts as the DMR in this imprinted region (Fig. 5), as mentioned in Theme 3. Importantly, the emergence of PEG10 corresponds the emergence of viviparity in mammals, thus leading to the concept of a “mammalian-specific exapted gene essential for a mammalian specific trait”. Actually, PEG10 is the first therian-specific gene exapted from an LTR retrotransposon/retrovirus shown to be critically involved in the establishment of the viviparous reproduction system common in therian mammals26-28.
The placenta is an organ facilitates oxygen/nutrient exchange between the mother and fetus(es). The eutherians have a sophisticated chorioallantoic placenta and deliver precocious young after long gestation periods, while marsupials have a simple choriovitelline (yolk sac) placenta and deliver altricial young after relatively short gestation periods. The placenta in the former is a derivative allantois and chorion and in the latter is comprised of a yolk sac and chorion. The allantois, yolk sac and chorion are three out of the four extraembryonic membranes unique to amniotes, a group that comprises reptiles, birds and mammals, which have another membrane, the amnion (Fig. 9). We believe that PEG10 was one of the key genes that triggered the change from the preexisting extraembryonic membranes to the placenta, and that the exaptation of PEG10 was one of the fundamental events in the evolutionary emergence of mammals.
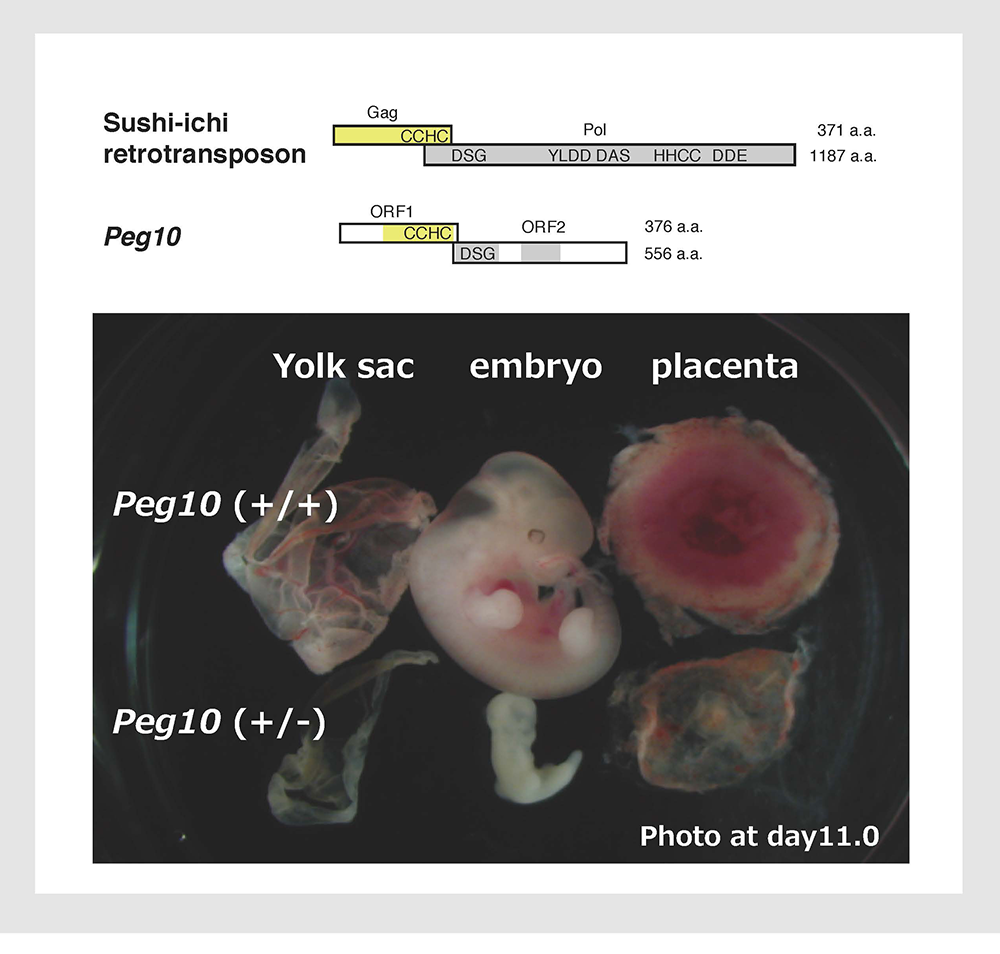
Peg10 KO mice exhibit early embryonic lethality due to severe placental defects.
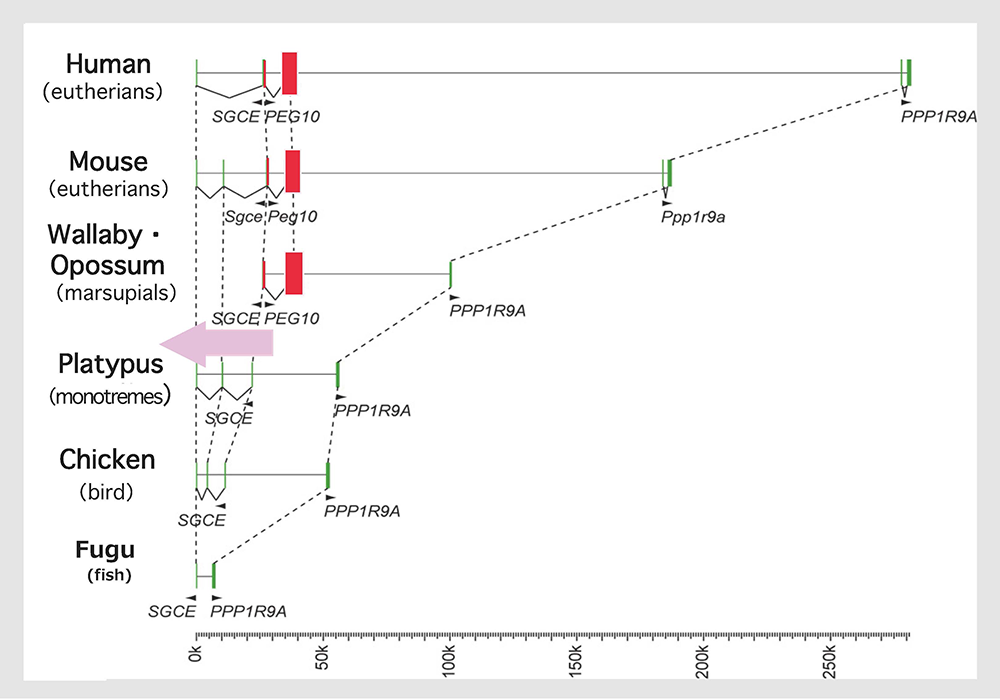
A comparison of the genome sequences of the higher vertebrates shows that the insertion of the original PEG10 retrotransposon/retrovirus occurred after the spilt of the therians and monotremes, as indicated by the pink arrow. Modified from Fig. 1 in ref. 24.
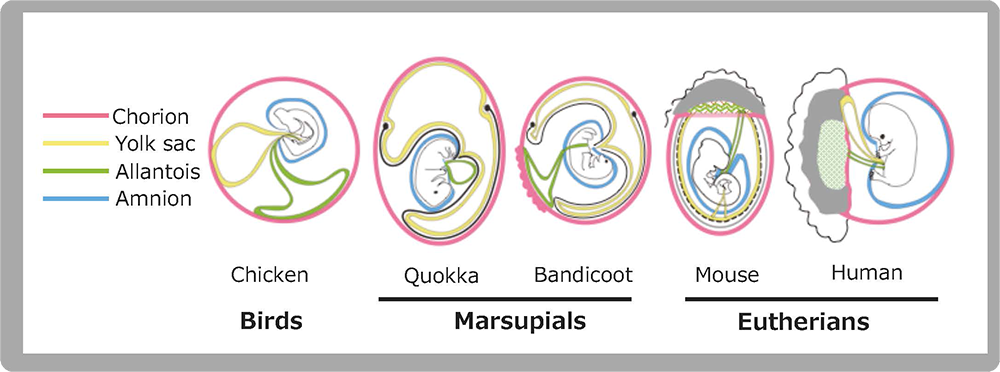
The amniotes (birds, reptiles and mammals) have 4 extraembryonic membranes (chorion (pink), yolk sac (yellow), allantois (green) and amnion (blue). Marsupials have a choriovitteline (yolk sac) placenta comprised of chorion and yolk sac membranes, while eutherians have a chorioallantoic placenta comprised of a chorion, allantois and trophoblast cells (gray) derived from the ectoplacental cone, indicating that mammalian placentas originated in such amniote-specific extraembryonic membranes. Two marsupial figures are modified from Fig. 7.17 in “Reproductive physiology of marsupials” by Tyndall-Biscoe and Renfree (1987).
Reference
- Ono R, Nakamura K, Inoue K, Naruse M, Usami T, Wakisaka-Saito N, Hino T, Suzuki-Migishima R, Ogonuki N, Miki H, Kohda T, Ogura A, Yokoyama M, Kaneko-Ishino T* and Ishino F*. Deletion of Peg10, an imprinted gene acquired from a retrotransposon, causes early embryonic lethality. Nat Genet 38, 101-106 (2006).
Theme 5: PEG11/RTL1 and SIRH/RTL are eutherian-specific exapted genes, and their functions are related to eutherian-specific traits
Maternal duplication of the distal chromosome 12 causes late stage embryonic/neonatal lethality associated with growth retardation (Fig. 2). We assumed that another imprinted gene essential for placental function would also exist in this imprinted region. By reading the entire genome sequence around Meg3/Gtl2, the first imprinted genes mapped in this region, we identified Peg9/Dlk1, Peg11, antiPeg11, Meg8 and Meg9 (Fig. 3). Among them, PEG11 (now called RTL1) exhibits homology to the sushi-ichi retrotransposon Gag and Pol, like PEG10. Georges’ group first reported the composition of imprinted genes in this region in sheep, including sheep PEG1131, and their result was basically the same as ours.
As expected, Peg11/Rtl1 KO mice exhibited late embryonic/neonatal lethality associated with growth retardation32 (Fig. 10) because the fetal capillary network in the labyrinth region of the placenta was destroyed in the KO placenta from mid- to late gestation32,33. The fetal capillary network is specific to the eutherian type of placenta, playing a critical role as the feto-maternal interface that exchanges oxygen/nutrients between the maternal and fetal blood (Fig. 9). The Ferguson-Smith and Renfree groups demonstrated that there exist no PEG11/RTL1 or MEGs in the orthologous marsupial chromosome, and there is no imprinting regulation in this region in marsupials. Thus, PEG11/RTL1 is the first eutherian-specific gene exapted from an LTR retrotransposon/retrovirus shown to be essential for the eutherian type of reproduction by maintaining placental function over the long gestation period26-28.
As mentioned, PEG10 and PEG11/RTL1 encode proteins exhibiting the highest homology to the sushi-ichi retrotransposon Gag and Pol and play a role as mammalian-specific exapted genes. Are there any other genes exapted from the same LTR retrotransposon/retrovirus in mammals? We screened genes exhibiting homology to the sushi-ichi retrotransposon Gag in both the human and mouse genomes and identified another 9 genes that we named “sushi-ichi retrotransposon related retrotransposon homologue (SIRH) 3-11 genes” with PEG10 and PEG11/RTL1 serving as SIRH1 and SIRH2, respectively30 (Fig. 11). They have also been called “mammalian-specific retrotransposon transcripts” (MART)35 as well as the “SUSHI family”36, but they are now formally termed retrotransposon Gag-like (RTL) genes, except for PEG10 and “leucine zipper, downregulated in cancer 1” (LDOC1, also called SIRH7 and RTL7). Only PEG10 is conserved in both marsupials and eutherians, while the other SIRH/RTL genes, PEG11/RTL1 and SIRH3-11, are eutherian-specific. Marsupials have their own SIRH12, which is absent from eutherians.
Most of the additional SIRH/RTL genes bear homology only to the Gag protein and encode relatively small proteins except for SIRH10/RTL9, while SIRH9/RTL3 shares homology with both the Gag and Pol proteins, like PEG10 and PEG11/RTL1 (Fig. 11). Are they functional? Except for the homology to Gag protein, we had no information on the biological functions of these genes, so the only way to answer this question was to make each KO mouse and elucidate the biological function(s) on a case-by-case basis. Thus far, our series of KO mouse analyses has demonstrated that at least 9 out of 11 SIRH/RTL genes have important functions in development and/or behavior, that is, they are actively functional in the placenta and/or brain (Figs. 11 and 12).
The placenta group is comprised of PEG10, PEG11/RTL1 and SIRH7/LDOC1/RTL7. The placenta is also known as a major endocrine organ during gestation, and in the case of mouse placenta, a variety of cells produce their own placental hormones to maintain pregnancy and determine the timing of parturition. The placentas in Sirh7/Ldoc1 KO embryos exhibited structural and related endocrinological problems, especially progesterone (P4) overproduction. The mice pregnant with such KO embryos exhibited delayed parturition. They also exhibited poor maternal care, leading to a low pup weaning rate37 (Fig. 12, also see Spin-off 1).
In the brain group, each KO mouse displayed unique behavioral abnormalities: Sirh11/Zcchc16/Rtl4 KO mice exhibit defects in adaptation to a new environment and increased impulsivity, presumably because of a low recovery rate of noradrenaline (NA) in the prefrontal cortex in the brain38 (see also Spin-off 2). Sirh3/Ldoc1l/Rtl6 KO mice exhibit increased vigilance and decreased daily activity (submitted), Sirh8/Rgag4/Rtl5 KO mice exhibit a low prepulse inhibition reaction that is widely observed in schizophrenia patients (unpublished data) and, in terms of the Sirh4-6/Rtl8cba triplet genes, Sirh5,6 DKO mice exhibit high aggressivity in males and frequent child neglect in females (unpublished data). As sophisticated brain function is also an important feature of eutherians, it is highly likely that these SIRH/RTL genes contributed to eutherian brain evolution after their diversification from marsupials.
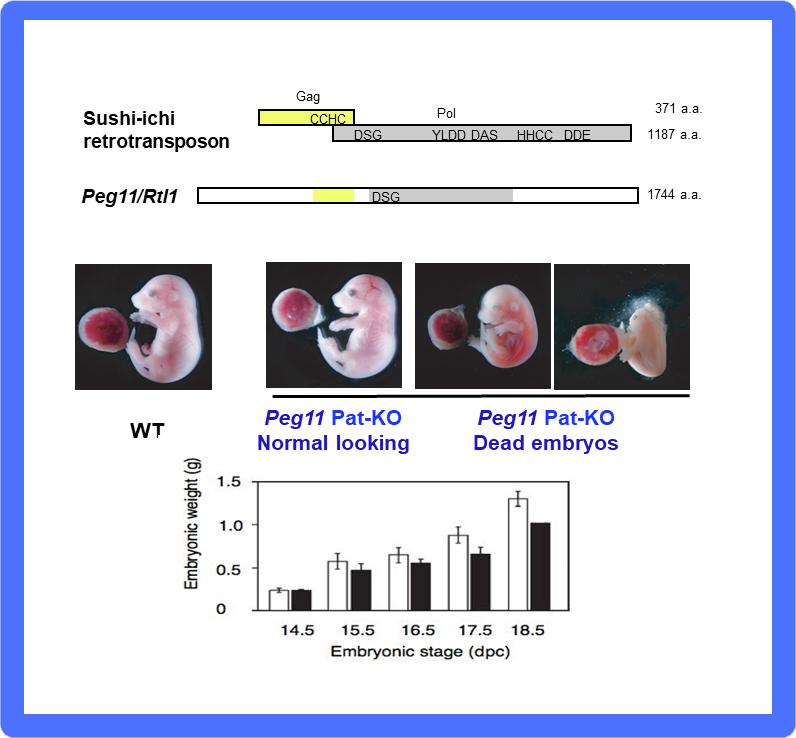
(Middle) One half of Peg11/Rtl1 KO mice exhibit late embryonic lethality and growth retardation due to severe defects in the fetal capillary network in the placenta. (Bottom) The other half also exhibit growth retardation and neonatal lethality within a day of birth.
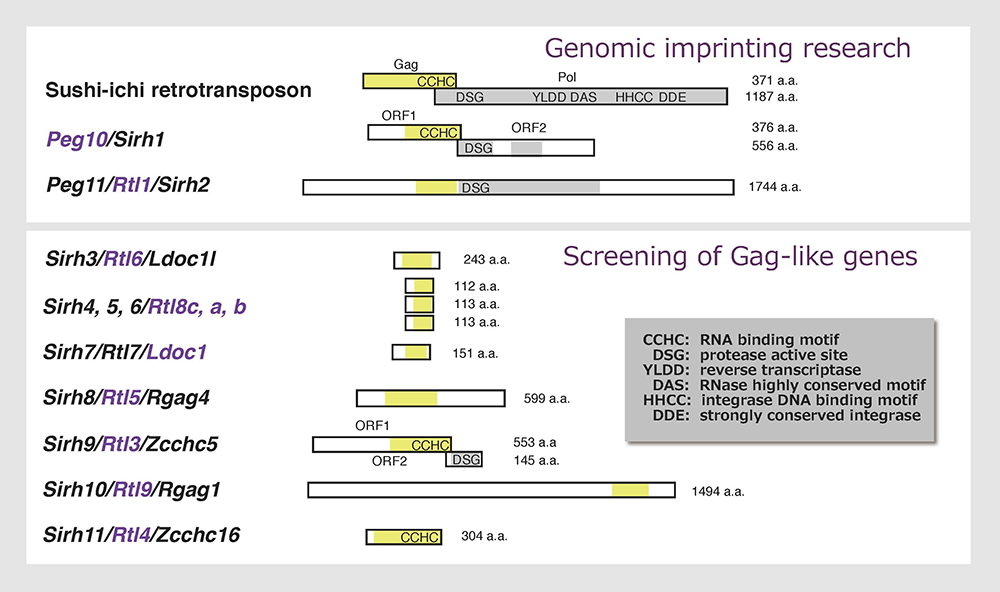
Mouse Sirh/Rtl genes are shown. They have 20~30 % homology with each other at the amino acid sequence level, suggesting each has its own particular biological function(s).
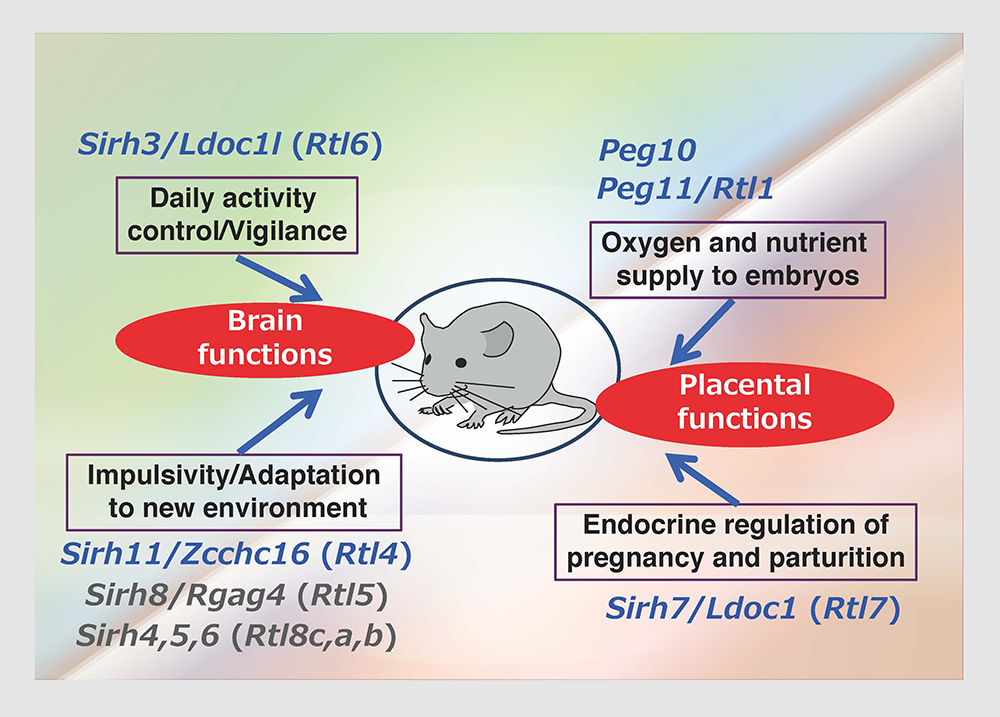
These genes are divided into two groups: one is a placenta group comprising Peg10, Peg11/Rgl1 and Sirh7/Ldoc1/Rtl7, the other is a brain group comprising Sirh3/Ldoc1l/Rtl6, Sirh4-6/Rtlc-a (possibly), Sirh8/Rgag4/Rtl5 and Sirh11/Zcchc16/Rtl4.
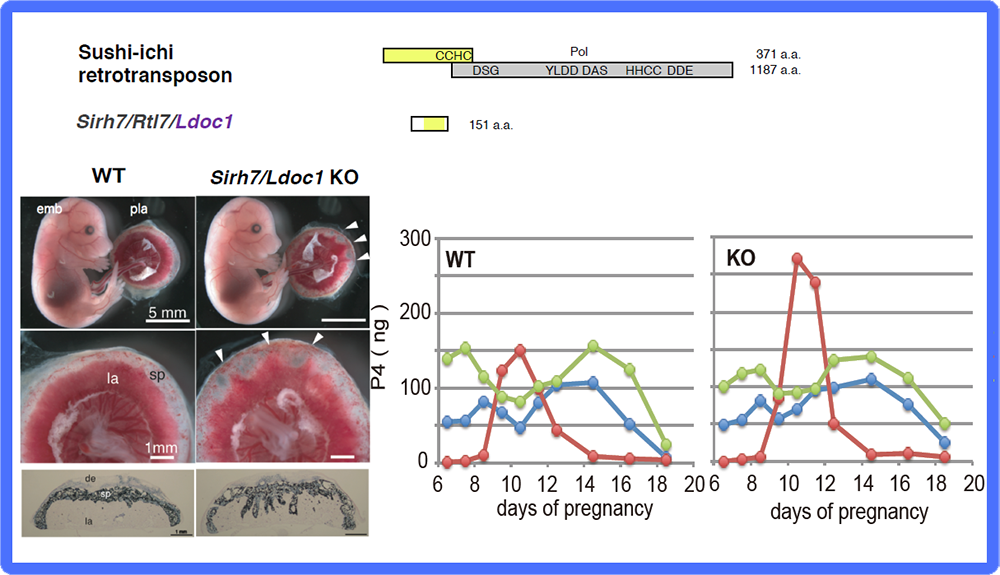
(Left) Fetuses and placentas on d15.5. The arrowheads indicate the regions where the labyrinth layers almost reached the maternal decidua. Despite the placental abnormalities, the Sirh7/Ldoc1 KO fetuses had a normal appearance and were born normally. (Right) Placental P4 production was observed mid-gestation when ovarian P4 production was temporally reduced, suggesting the importance of placental P4 production in mice. Overproduction of placental P4 was observed in Sirh7/Ldoc1 KO mice, leading to delayed parturition of pregnant females associated with a low pup weaning rate.
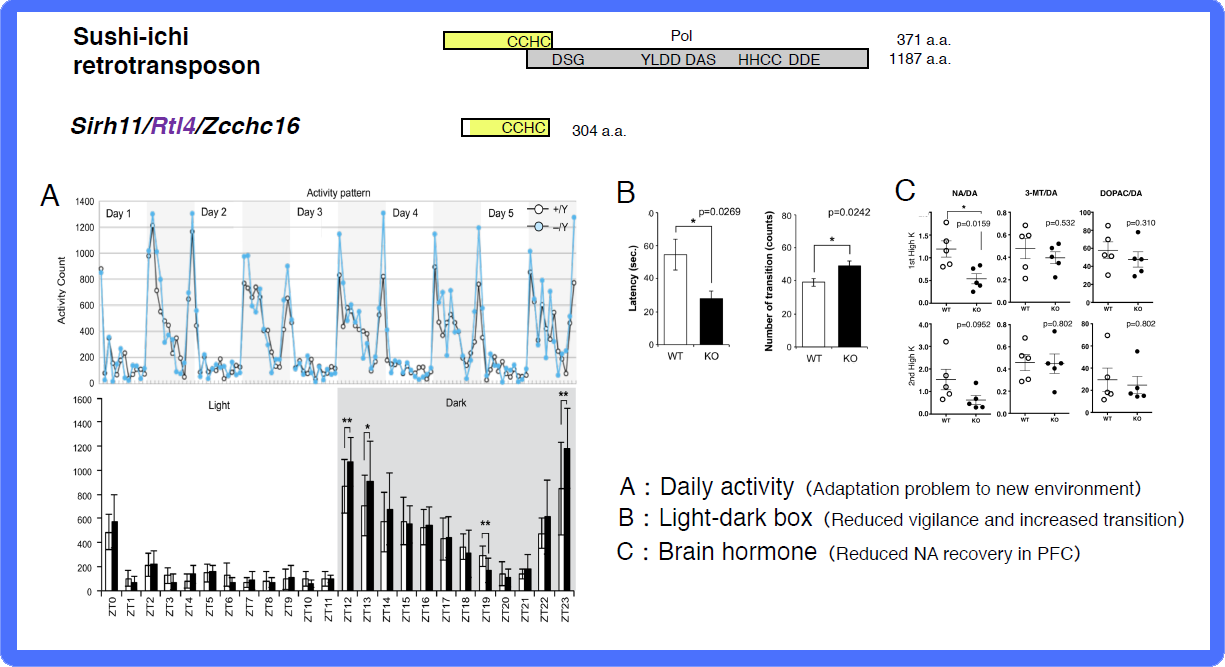
A: Home-cage activity test. (Top) The activity counts every hour over 5 days. The white and grey areas indicate the light and dark phases, respectively. (Bottom) The white and black bars represent the activity counts in the WT and KO mice, respectively. Zeitgeiber time (ZT) is shown on the x-axis. B: Light/Dark transition test. The left panel shows that KO mice exhibit a shorter latency time before entering into the light chamber. The right panel shows that the number of transitions is increased in KO mice. The white and black bars represent the WT and KO mice, respectively. C: Microdialysis analysis in the prefrontal cortex in the cerebrum. The recovery levels of DA and NA are shown after a perfusion of high potassium-containing artificial cerebrospinal fluid.
References
- Smits G, Mungall A J, Griffiths-Jones S, Smith P, Beury D, Matthews L, Rogers J, Pask A J, Shaw G, VandeBerg J L, McCarrey J R, SAVOIR Consortium, Renfree M B, Reik W and Dunham I. Conservation of the H19 noncoding RNA and H19-IGF2 imprinting mechanism in therians. Nat Genet 40, 971-976 (2008).
- Sekita Y, Wagatsuma H, Nakamura K, Ono R, Kagami M, Wakisaka N, Hino T, Suzuki-Migishima R, Kohda T, Ogura A, Ogata T, Yokoyama M, Kaneko-Ishino T*and Ishino F*. Role of retrotransposon-derived imprinted gene, Rtl1, in the feto-maternal interface of mouse placenta. Nat Genet 40, 243-248 (2008).
- Kitazawa M, Tamura M, Kaneko-Ishino T* and Ishino F*. Severe damage to the placental fetal capillary network causes mid to late fetal lethality and reduction of placental size in Peg11/Rtl1 KO mice. Genes Cells 22, 174-188 (2017).
- Edwards C A, Mungall A J, Matthews L, Ryder E, Gray D J, Pask A J, Shaw G, Graves J A, Rogers J, SAVOIR consortium, Dunham I, Renfree M B, Ferguson-Smith A C. The evolution of the DLK1-DIO3 imprinted domain in mammals. PLoS Biol 6, e135 (2008).
- Brandt J, Schrauth S, Veith A M, Froschauer A, Haneke T, Schultheis C, Gessler M, Leimeister C and Volff J N. Transposable elements as a source of genetic innovation: expression and evolution of a family of retrotransposon-derived neogenes in mammals. Gene 345, 101-111 (2005).
- Youngson N A, Kocialkowski S, Peel N and Ferguson-Smith A C. A small family of sushi-class retrotransposon-derived genes in mammals and their relation to genomic imprinting. J Mol Evol 61, 481–490 (2005).
- Naruse M, Ono R, Irie M, Nakamura K, Furuse T, Hino T, Oda K, Kashimura M, Yamada I, Wakana S, Yokoyama M, Ishino F* and Kaneko-Ishino T*. Sirh7/Ldoc1 knockout mice exhibit placental P4 overproduction and delayed parturition. Development 141, 4763-4771 (2014).
- Irie M, Yoshikawa M, Ono R, Iwafune H, Furuse T, Yamada I, Wakana S, Yamashita Y, Abe T, Ishino F* and Kaneko-Ishino T*. Cognitive function related to the Sirh11/Zcchc16 gene acquired from an LTR retrotransposon in eutherians. PLoS Genet 11:e1005521 (2015).
- Ono R, Kuroki Y, Naruse M, Ishii M, Iwasaki S, Toyoda A, Fujiyama A, Shaw G, Renfree M B, Kaneko-Ishino T* and Ishino F*. Identification of SIRH12, a retrotransposon-derived gene specific to marsupial mammals. DNA Res 18, 211-219 (2011).
Theme 6: Biological evolution in mammals: perspectives on genomic imprinting as well as mammalian-specific genes exapted from LTR retrotransposon/retrovirus
Why has genomic imprinting been so widely conserved in mammals? Genomic imprinting is seemingly contradictory to the Darwinian theory of evolution because of its apparent developmental disadvantage. It is clear that in diploid organisms biallelic expression is better than monoallelic expression, especially in genes essential for development. Living without any safeguard is likely to endanger the survival of individuals in both the pre- and postnatal periods. However, what are we to make of a situation in which PEGs and MEGs cannot be expressed from single chromosomes because of the insertion of foreign DNA? As we discussed in the Theme 3 section, it is probable that genomic imprinting arose as a genome defense mechanism against the insertion of foreign DNA sequences with certain cis-acting activity, resulting in a reciprocal and complementary (this inevitably means monoallelic) expression system of PEGs and MEGs from two different parental chromosomes. This idea is supported by the fact that each DMR is comprised of a different newly inserted sequence24-28. In such a case, the parent-of-origin monoallelic expression of the PEGs and MEGs from the two parental chromosomes would come to be essential for survival, that is, it would confer an apparent advantage on the organism. This is our answer to the fundamental question about why genomic imprinting has been so widely conserved despites its apparent developmental disadvantage, and we have proposed this idea as “the complementation hypothesis”17,27,28. In other word, genomic imprinting is an evolutionary trade-off for survival over and against the disadvantageous monoallelic expression of several essential and/or important developmental genes27,28. The genome defense hypothesis was independently proposed by Dense Barlow in 199340. Her idea is basically the same as the first part of our hypothesis, but differs in that our hypothesis, coupled with the complementation hypothesis, proposes an essential nature for the genomic imprinting in the mammalian developmental system. In summary, we think it highly probable that genomic imprinting was first established as an essential mechanism for survival in mammals by the complementation of the two parental genomes and as an adaptation of the viviparous reproduction system despite the conflict between the two parental genomes that arose in mammalian evolution.
Mammalian-specific genes exapted from LTR retrotransposons/retroviruses, such as PEG10, PEG11/RTL1 and SIRH3-11, provide good examples of macroevolution in mammals because it is apparent that after their exaptation they were positively selected due to the advantage conferred on eutherian reproductive success27,41,42 (Fig. 15A and B). This scheme is in accord with the process of “competition among individuals within the same species” in the Darwinian theory of evolution. Each of eutherian specific SIRH/RTL genes follows the scheme shown in Fig. 15B. However, a new question arises when we take into account the number of exaptation events (one in therians and 10 in eutherians). How could the common eutherian ancestor have acquired that many exapted genes? Were they acquired sequentially in a single line (Fig. 16A) or were they unified from multiple lines (Fig. 16B)? Both schemes are possible and it would have depended on the specific situation. In the Darwinian theory of evolution, competitive success via advantage arising in a single individual tends to be emphasized. If such events occurred repeatedly, these exapted genes may have come to be sequentially inserted in a single eutherian ancestral line, as shown in Fig. 16A. Another possibility is that all of the exapted genes may have been accumulated in a single line by sexual mating across multiple generations of individuals with one or a small number of exapted genes, thus leading to the common eutherian ancestor(s), as shown in Fig. 16B42. The latter scheme also fits with Darwin’s theory because each advantageous gene was selected for the biological advantage it conferred, as shown in Fig. 15B. This is a reminder that the presence of multiple individuals, namely the group scale, is relevant to the accumulation of a variety of advantageous mutations in the same group. In other word, the diversity among the individuals within a given group is a powerful source of the future success of the organism. Thus, “cooperation among individuals within the same species” in the form of the interactive behaviors of the individuals within a tightly delimited species is also a critically important process in biological evolution in addition to “competition among individuals within the same species”.
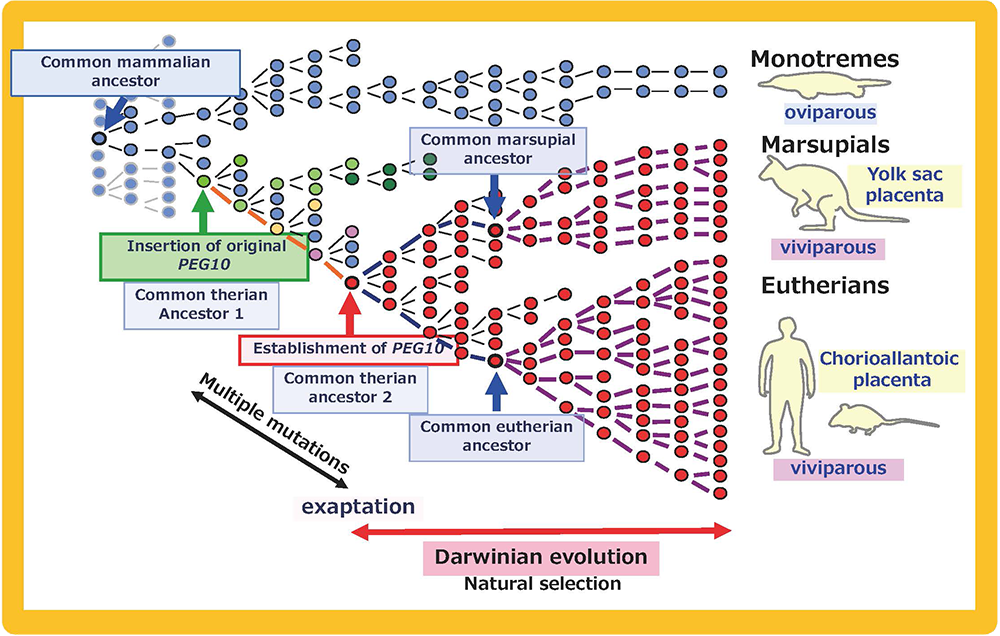
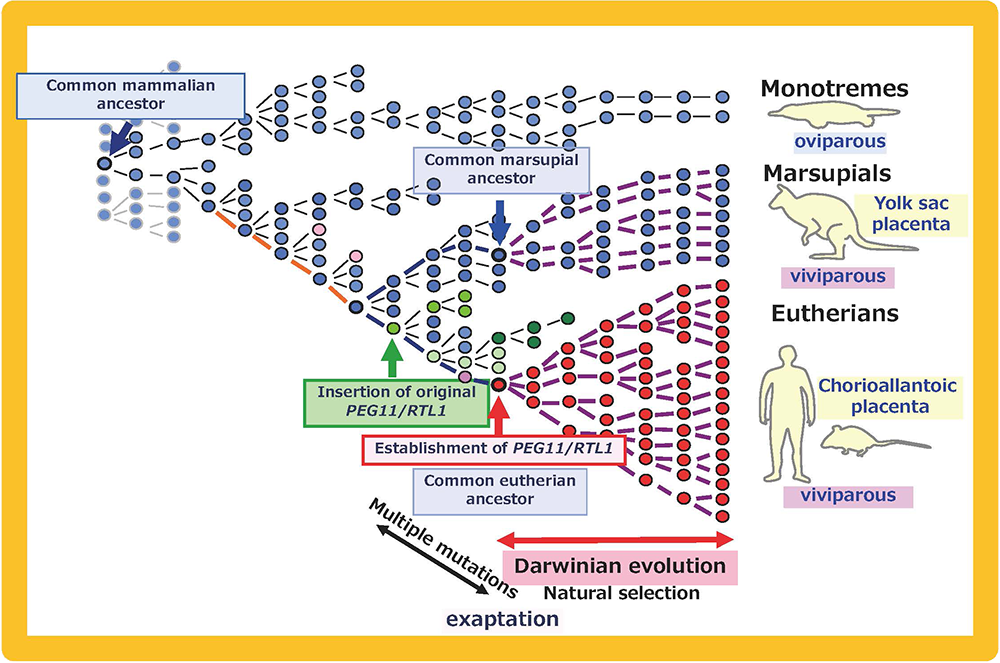
A: PEG10, Integration of the original PEG10 retrotransposon/retrovirus (green arrow) occurred after the divergence of the monotremes and the therian mammals. After its exaptation (red arrow), PEG10 was positively selected and propagated into all the eutherians and marsupials. Multiple mutations might have been required for the establishment of PEG10 function. B: PEG11/RTL1, The integration of the original PEG11/RTL1 retrotransposon/retrovirus (green arrow) occurred after the divergence of the eutherians and marsupials. After its exaptation (red arrow), PEG11/RTL1 was positively selected and propagated into all the eutherians. Multiple mutations might have been required for the establishment of PEG11/RTL1 function. This scheme also fits other SIRH/RTL genes, although some lost their function in a lineage-specific manner.
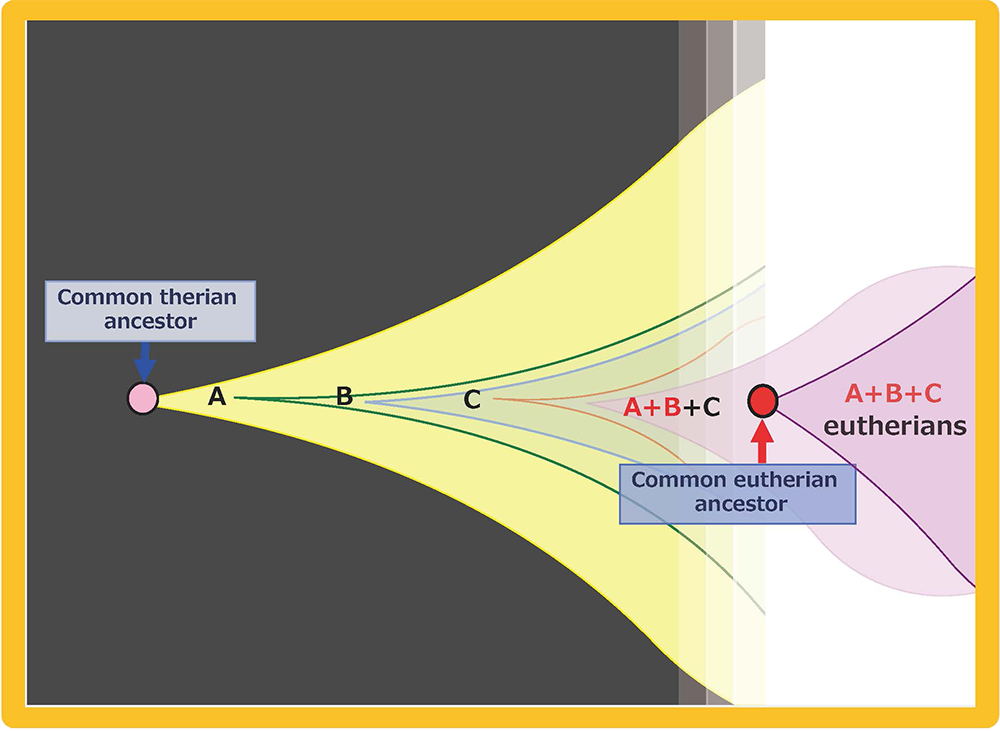
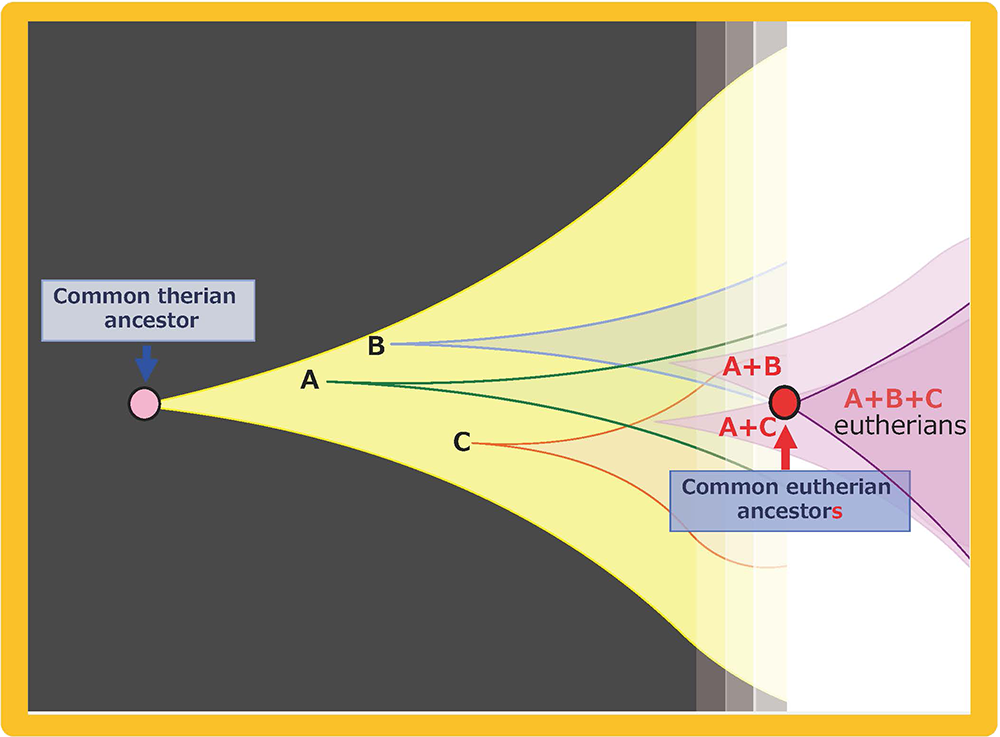
Multiple exaptations may have occurred by (A) sequential acquisition in a single ancestral line and/or (B) multiple acquisitions in parallel lines, followed by unification into a single ancestral line by sexual mating. Both of these scenarios fit the Darwinian theory of evolution. We see “competition among individuals in the same species” in both, however, we also see “cooperation among individuals in the same species” in the latter, indicating that the integrated activities of a number of individuals or lines within the same species might have played an essential background role in generating the common eutherian ancestral line.
References
- Barlow D P. Methylation and imprinting: from host defense to gene regulation? Science 260, 309-310 (1993).
- Kaneko-Ishino T and Ishino F. Retrotransposon silencing by DNA methylation contributed to the evolution of placentation and genomic imprinting in mammals. Develop Growth Differ 52, 533-543 (2010).
- Kaneko-Ishino T and Ishino F. Cooperation and Competition in Mammalian Evolution -Gene Domestication from LTR Retrotransposons- In Evolution, Origin of Life, Concepts and Methods, Chapter 15 (Ed. Pontarotti P), Springer Nature, pp.317-333 (2019).
7. PEG11/RTL1 contributions to muscle development and brain functions in the course of eutherian evolution.
In Theme 5, we showed that Peg11/Rtl1 plays an essential role in the maintenance of placental function in mice, thereby, presumably contributing to the evolution of the eutherian placenta. Moreover, Peg11/Rtl1 plays not only an essential role in muscle development42 in a fetal/neonatal stage-specific manner, but also plays several important roles in the nervous system43 in mice, suggesting that human PEG11/RTL1 is the major gene responsible for the Kagami-Ogata and Temple syndromes (KOS14 and TS14, respectively). These two imprinted diseases are caused by paternal and maternal disomy of chromosome 14, which is orthologous to mouse chromosome 12, where Peg11/Rtl1 is located. KOS14 patients exhibit neonatal lethality, presumably due to the respiratory problems associated with a small bell-shaped thorax, abdominal wall defects, such as omphalocele and diastasis recti, placentomegaly, polyhydramnios, developmental delay and/or cognitive disability and feeding disorders. TS14 patients exhibit severe pre- and postnatal growth retardation, hypotonia, early onset of puberty, motor delay, feeding difficulties and mild intellectual disabilities. Overexpression and deficiency (or under-expression in the case of the brain) of PEG11/RTL1 are considered to be the cause of the above mentioned placental, muscle and brain defects in KOS14 and TS14.
In the fetal/neonatal muscles, the PEG11/RTL1 protein is co-localized with DESMIN, a sarcomeric cytoskeleton that displays certain links between membranes and sarcomeres at the Z-disc44. Interestingly, Desmin KO mice exhibit lethality approximately 2 weeks after birth, the same timing as the disappearance of PEG11/RTL1 in the muscles45, suggesting that PEG11/RTL1 functions instead of DESMIN and/or represses the DESMIN function in the fetal/neonatal periods.
In the neonatal brain, PEG11/RTL1 is expressed in the pyramidal tracts from cerebral cortex layer V to the spinal cord, including the corticospinal, corticonuclear and corticoreticular tracts, interhemispheric neurons including the corpus callosum, and the limbic system. It is also expressed in corticothalamic tract from cerebral cortex layer VI, suggesting its involvement in sensory processing in addition to motor function. The corticonuclear tract enervates the cranial nerves and the corticoreticular tract regulates the spinal nerves via reticulospinal tract. These results indicate that the KOS14 and TS14 are neuromuscular as well as neuropsychiatric diseases caused by irregular CNS RTL1 expression. It is known that the corticospinal tract and corpus callosum are mammalian- and eutherian-specific brain structures. It is highly likely that the emergence of the corpus callosum was also involved in the functional superiority of the eutherian corticospinal tract system compared to the marsupial one46, 47.
Thus, despite its evolutionary origin as an extinct retrovirus, PEG11/RTL1 is considered to critically contribute to multiple eutherian developmental functions: 1) the maintenance of the placental fetal capillary network required for the long gestation period, 2) the functional enhancement of motor and sensory neurons (layer V and VI, respectively), interhemispheric neurons and the limbic system and 3) the regulation of fetal/neonatal muscles for the protection both the fetus and mother. In the context of eutherian evolution, RTL1 must have been involved in the emergence of the following. 1) The eutherian type placenta needed for the long gestational period in the eutherian reproduction system and 2) the eutherian type of brain with its greatly enhanced nervous system as well as 3) the fetal/ neonatal adaptation to the long gestational and weaning periods that supported these changes.
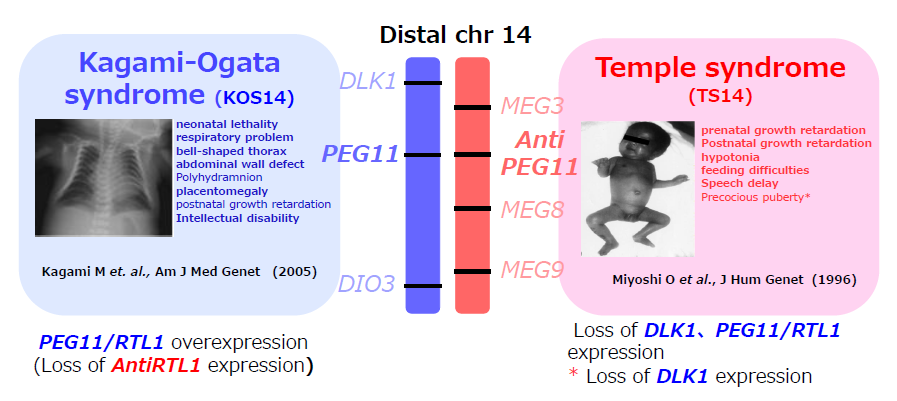
Paternal and maternal disomy of human chromosome 14 causes Kagami-Ogata and Temple syndromes (KOS14 and TS14, respectively). This imprinted region is comprised of three protein-coding PEGs (DLK1, PEG11/RTL1, DIO3) and four MEGs of non-coding RNAs (MEG3, AntiPEG11/antiRTL1, MEG8, MEG9). In the usual case of paternal disomy, PEG expression is doubled and MEG expression is lost. However, because of the loss of the six microRNAs encoded in AntiPEG11/antiRTL1 that target complementary PEG11/RTL1 mRNA, PEG11/RTL1 expression increases 4- to 6-fold. This overexpression of PEG11/RTL1 is considered to cause most of the defects in the placenta, muscle and brain in the case of KOS 14. In contrast, deficiency and/or under-expression (in the brain) of PEG11/RTL1 is considered to cause most of the defects in TS14 coupled with the loss of DLK1, except for the early onset of puberty caused by the loss of DLK1.
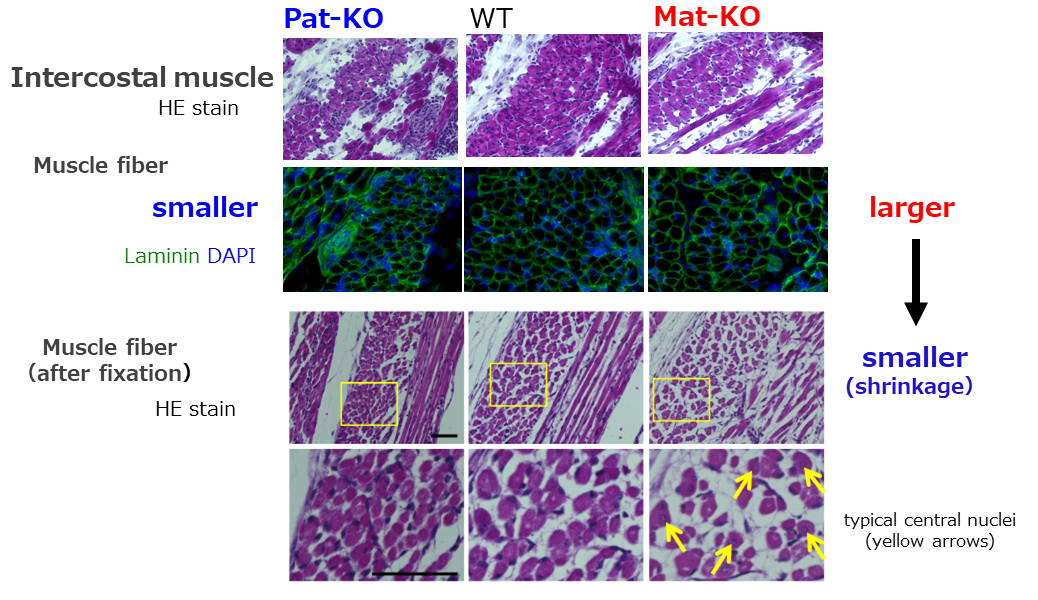
The neonatal intercostal muscles of cases of deficiency and overexpression of PEG11/RTL1 (left and right, respectively) and the (of) control wild type (middle). The images of the HE (staining) and immunofluorescence staining of Laminin (green) and DAPI (blue) are shown. The deficiency and overexpression of PEG11/RTL1 cause thinner and large muscle fibers, respectively (top two columns). However, severe shrinkage occurred in the latter under a fixed condition (bottom two columns). The arrows in the latter indicate muscle fibers with centrally-located nuclei, indicating the immaturity of the muscle cells.
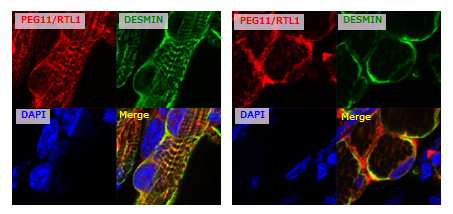
Immunofluorescence staining of PEG11/RTL1 (red, top left) and DESMIN (green, top right) proteins in the neonatal forelimb muscles (from) mice with overexpression of PEG11/RTL1. Long axis views (left) and cross-sectional views (right) of the muscle fibers. The nuclei are stained with DAPI (blue, bottom left), and their merged images are shown (bottom right).
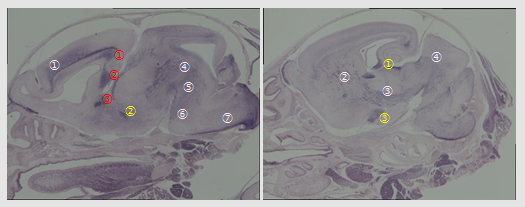
Immunostaining of PEG11/RTL1 in P0 neonatal brain. 1) Corticospinal tract (numbers in white); from the fifth layer of cerebral cortex to spinal cord. ① layer V: the fifth layer of cerebral cortex, ② IC: internal capsule, ③ CP: cerebral peduncle, ④ Mb: midbrain, ⑤ Hb: hindbrain, ⑥ Po: pons, ⑦ MO: medulla oblongata. 2) Interhemispheric projection neurons (numbers in red); ① CC: corpus callosum, ②HC: hippocampal commissure and ③ AC: anterior commissure. 3) Limbic system (numbers in yellow); ① HF: hippocampal fimbria, ② Fx: fornix and ③ MEA: Medial amygdala nucleus.
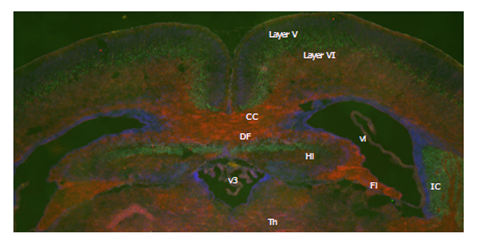
A coronal section of the P0 neonatal brain. Immunofluorescent staining of RTL1 (red), CTIP2 (green), a marker of the fifth layer, and DAPI (blue). CC: corpus callosum, DF: dorsal fornix, Fi: fimbria, Hi: hippocampus, IC: internal capsule, layer V: the fifth layer of cerebral cortex, layer VI: the sixth layer of cerebral cortex, Th: thalamus, v3: the 3rd ventricle, vl: lateral ventricle.
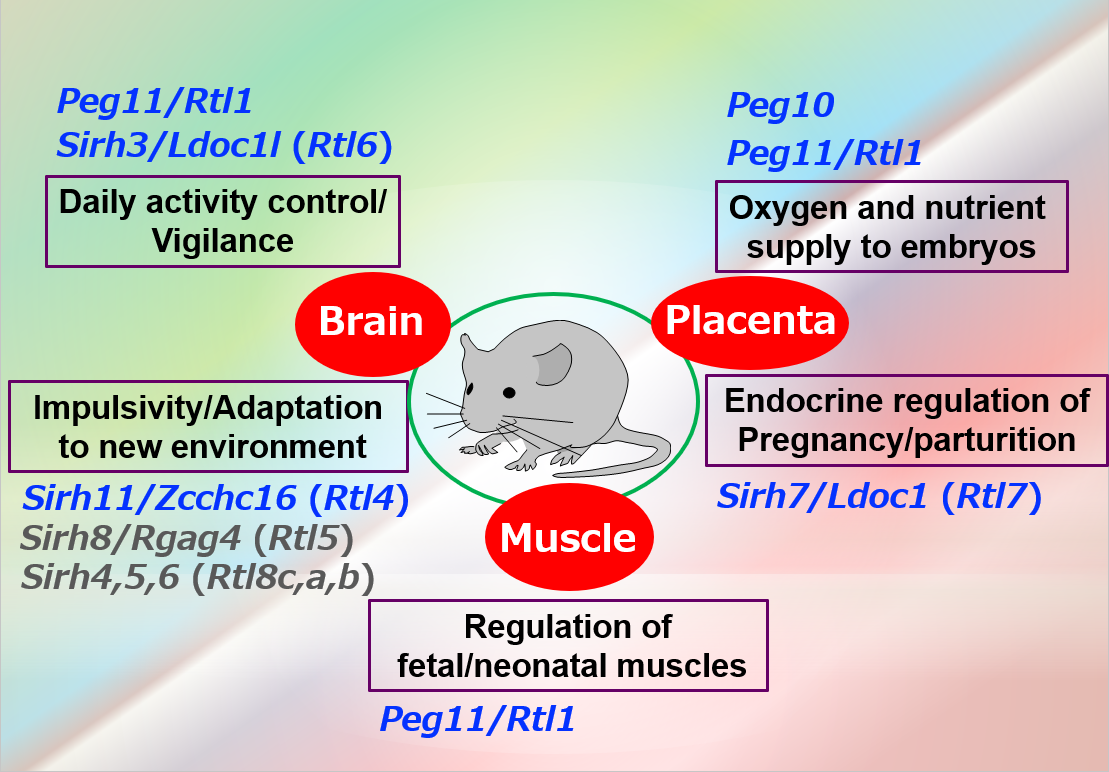
References
- Kitazawa M, Hayashi S, Imamura H, Takeda S, Oishi Y, Kaneko-Ishino T and Ishino F. Deficiency and overexpression of Rtl1 in the mouse cause distinct muscle abnormalities related to the Temple and Kagami-Ogata syndromes. Development 147:dev185918 (2020) doi: 10.1242/dev.185918.
- Kitazawa M, Sutani A, Kaneko-Ishino T and Ishino F. The role of eutherian-specific RTL1 in the nervous system and its implications for the Kagami-Ogata and Temple syndromes. Genes Cells (2021) doi: 10.1111/GTC.12830
- Goldfarb LG and Dalakas MC. Tragedy in a heartbeat: malfunctioning desmin causes skeletal and cardiac muscle disease. J Clin Invest 119, 1806-1813 (2009).
- Milner DJ, Weitzer G, Tran D, Bradley A and Capetanaki Y. Disruption of muscle architecture and myocardial degeneration in mice lacking desmin. J Cell Biol 134, 1255-1270 (1996).
- Ivanco TL, Pellis SM and Whishaw IQ. Skilled forelimb movements in prey catching and in reaching by rats (Rattus norvegicus) and opossums (Monodelphis domestica) relations to anatomical differences in motor systems. Behavior Brain Res, 79, 163–181 (1996).
- Frost SB, Milliken GW, Plautz EJ, Masterton RB and Nudo RJ. Somatosensory and motor representations in cerebral cortex of a primitive mammal (Monodelphis domestica): A window into the early evolution of sensorimotor cortex. J Comp Neurol, 421, 29–51 (2000).
Theme 8: Genes expressed in neurons that regulate brain function— Sirh4, 5, 6/Rtl8c, a, b—
Among the SIRH/RTL genes functioning in neurons, PEG11/RTL1is known as the causative gene for Kagami-Ogata syndrome and Temple syndrome (Theme 7). Recently, PEG10 has also been found to be expressed in neurons, and its involvement in human diseases, Angelman syndrome (AS) and Amyotrophic Lateral Sclerosis (ALS), has been suggested1,2. Additionally, our research has also revealed significant abnormalities in brain-specific Peg10 KO mice (Shiura, unpublished data).
This group of neuron-functional genes also includes Sirh4, 5, 6/Rtl8c, a, b, which exist as a cluster of three homologous genes on the X chromosome3. In eutherians, including humans, they are typically found as a three-gene cluster, but their biological function and the reason for their triplicate existence have remained unclear for a long time. Knocking out Sirh4/Rtl8c alone did not result in any noticeable abnormalities (our unpublished data). However, double knockout (DKO) mice lacking both Sirh5, 6/Rtl8a, b exhibited increased food intake and a tendency toward obesity from early adulthood (around week 8)3 (Figure 23). Additionally, half of the heterozygous DKO female mice and all of the homozygous DKO female mice exhibited severe deficits in maternal nursing behavior, resulting in the near-complete loss of their offspring. When the offspring were raised by foster mothers, they developed normally, confirming that the issue lay with the DKO mothers (Figure 24). These findings suggest that Sirh5, 6/Rtl8a, b are crucial genes for eutherian mammals, playing a vital role in maternal nursing behavior3. Comprehensive behavioral analyses revealed motor dysfunction, reduced sociability, and increased apathy, indicating abnormal brain function3 (Figure 25).
These symptoms, along with late-onset obesity, resemble the manifestations of late-stage Prader-Willi syndrome (PWS), a genomic imprinting disorder caused by maternal uniparental disomy of chromosome 15q11-q134. Recently, it was reported that iPS cells derived from patients with AS—a neurodevelopmental disorder caused by paternal uniparental disomy of the same chromosome 15q11-q13, associated with the loss of expression of the maternally expressed imprinted gene UBE3A (ubiquitin E3 ligase A)4—accumulate excessive RTL8 and PEG10 proteins when differentiated into neurons1. UBE3A functions as a ubiquitin ligase enzyme, and this finding suggests that it directly targets RTL8 and PEG10 proteins for degradation. Given this, in PWS, UBE3A expression is elevated, likely leading to a reduction in RTL8 protein levels. This aligns well with our observations in Sirh5, 6/Rtl8a, b double knockout (DKO) mice, which resemble the molecular condition seen in PWS. Notably, PWS-like symptoms have also been reported in cases of Xq26-q28 inversion involving RTL85. Currently, we are investigating the potential link between this disorder and the Sirh4, 5, 6/Rtl8c, a, b genes3
Note:
Capital alphabet italicized letters like RTL8A, B, and C represent human genes, while only the first letter is capital italicized for mouse like Rtl8a, b, and c. Capital solid letters RTL8A, B, and C represent proteins without distinction between human and mouse.
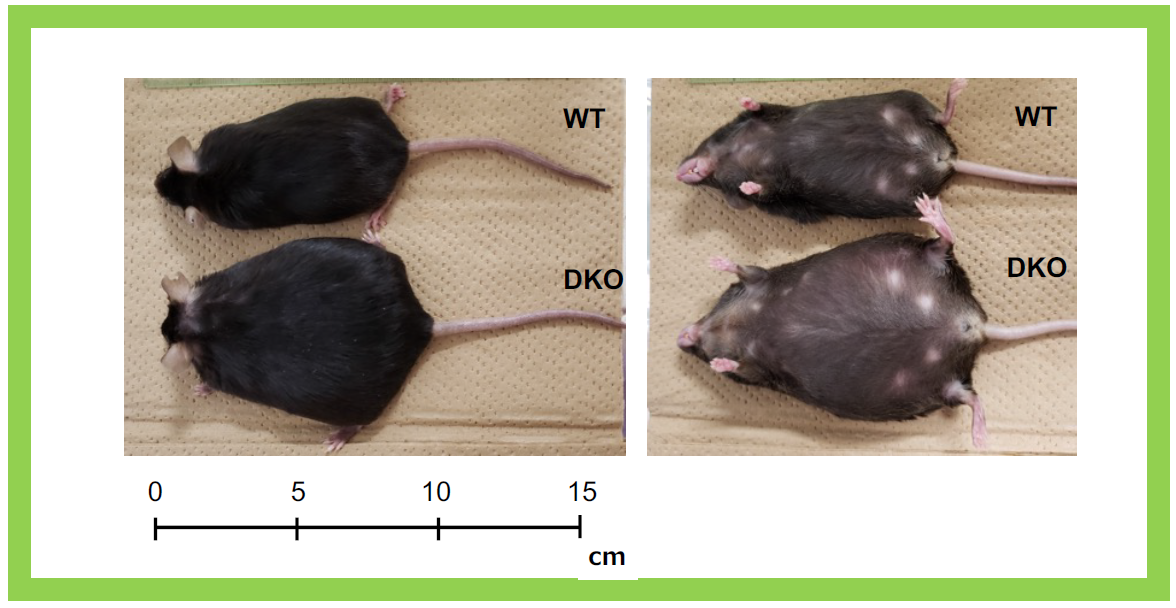
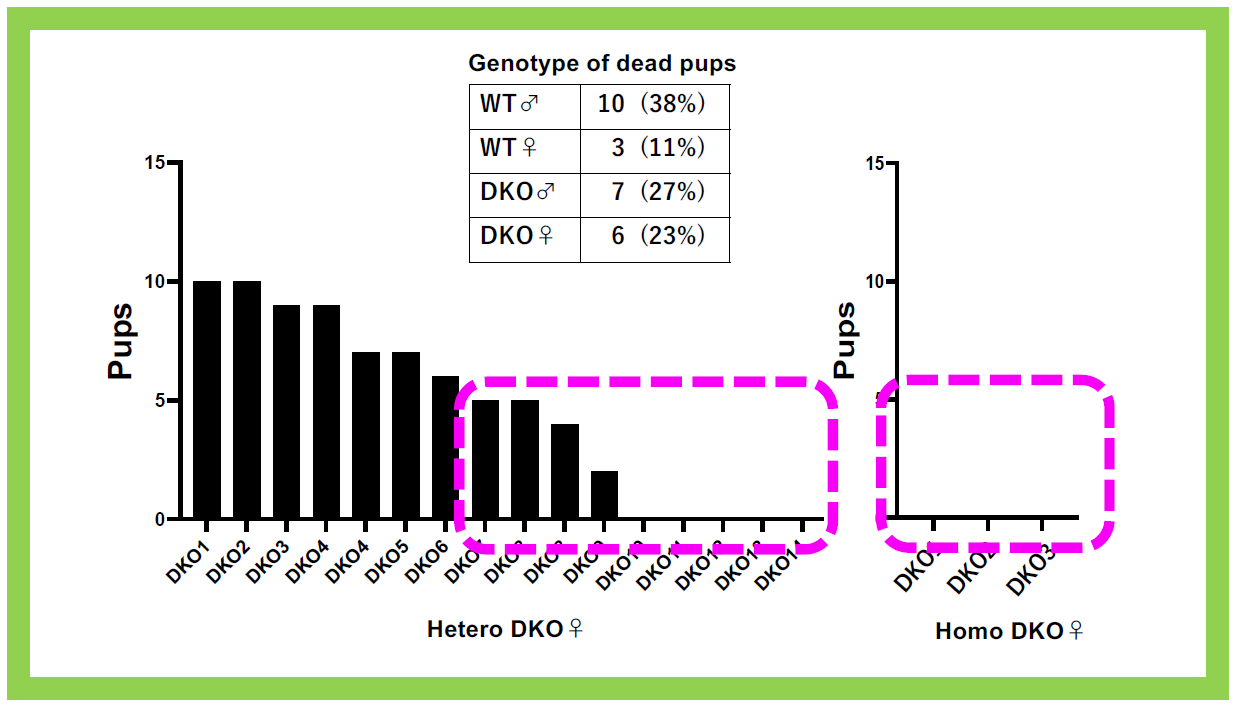
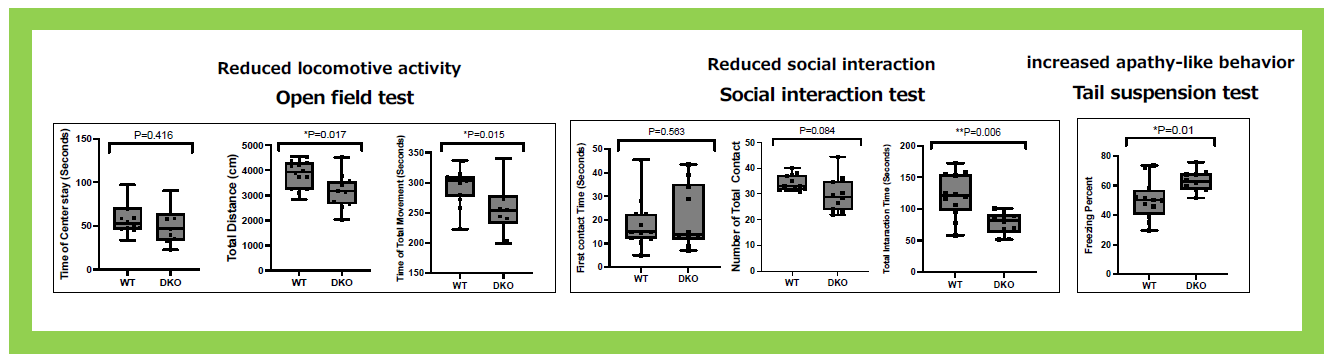
- Pandya NJ, Wang C, Costa V, Lopatta P, Meier S, Zampeta FI, Punt AM, Mientjes E, Grossen P, Distler T et al. Secreted retrovirus-like GAG-domain-containing protein PEG10 is regulated by UBE3A and is involved in Angelman syndrome pathophysiology. Cell Rep Med 2:100360 (2021).
- Black HH, Hanson JL, Roberts JE, Leslie SN, Campodonico W, Ebmeier CC, Holling GA, Tay JW, Matthews AM, Ung E, Lau CI and Whiteley AM. UBQLN2 restrains the domesticated retrotransposon PEG10 to maintain neuronal health in ALS. Elife12:e79452 (2023).
- Fujioka Y, Shiura H, Ishii M, Ono R, Endo T, Kiyonari H, Hirate Y, Ito H, Kanai-Azuma M, Kohda T, Tomoko Kaneko-Ishino T and Ishino F. Targeting of retrovirus-derived Rtl8a/8b causes late-onset obesity, reduced social response and increased apathy-like behavior. Open Biol 15: 240279 (2025).
- Nicholls RD and Knepper JL. Genome organization, function, and imprinting in Prader-Willi and Angelman syndromes. Annu Rev Genomics Hum Genet 2, 153–175 (2001).
- Florez L, Anderson M and Lacassie Y. De novo paracentric inversion (X)(q26q28) with features mimicking Prader–Willi syndrome. Am J Med Genet 121A, 60–64 (2003).
Theme 9:Genes expressed in microglia, the immune cells of the brain—Sirh3, Sirh8, Sirh10 and Sirh11—
Innate immunity is a fundamental biological defense system, widely conserved across the animal kingdom. When pathogens or foreign substances invade the body, phagocytic cells such as macrophages and neutrophils respond quickly to eliminate them. In the nervous system, microglia perform this role. They are capable of responding rapidly to a wide range of foreign substances through multiple Toll-like receptors (TLRs), which recognize specific foreign substances and induce inflammatory responses. These foreign substances, known as pathogen-associated molecular patterns (PAMPs), are unique molecular structures specific to pathogens. For example, lipopolysaccharide (LPS) from the cell walls of Gram-negative bacteria, dsRNA or unmethylated DNA from viruses, and zymosan from fungal cell walls are recognized by TLR7, TLR3, and TLR2/6 proteins, respectively (Figure 26).
Within the SIRH/RTL gene family, four genes are specifically expressed in microglia, playing a crucial role in protecting the brain in eutherian mammals. Three of these genes directly bind to PAMPs, facilitating their rapid elimination and contributing significantly to infection defense (Figure 26). The remaining gene responds to mental stress related to noradrenaline and is associated with a neurodevelopmental disorder (Figures 33 and 34).
9-1 Natural immune defenders of the brain against infection: Sirh3/Rtl6, Sirh8/Rtl5 and Sirh10/Rtl9
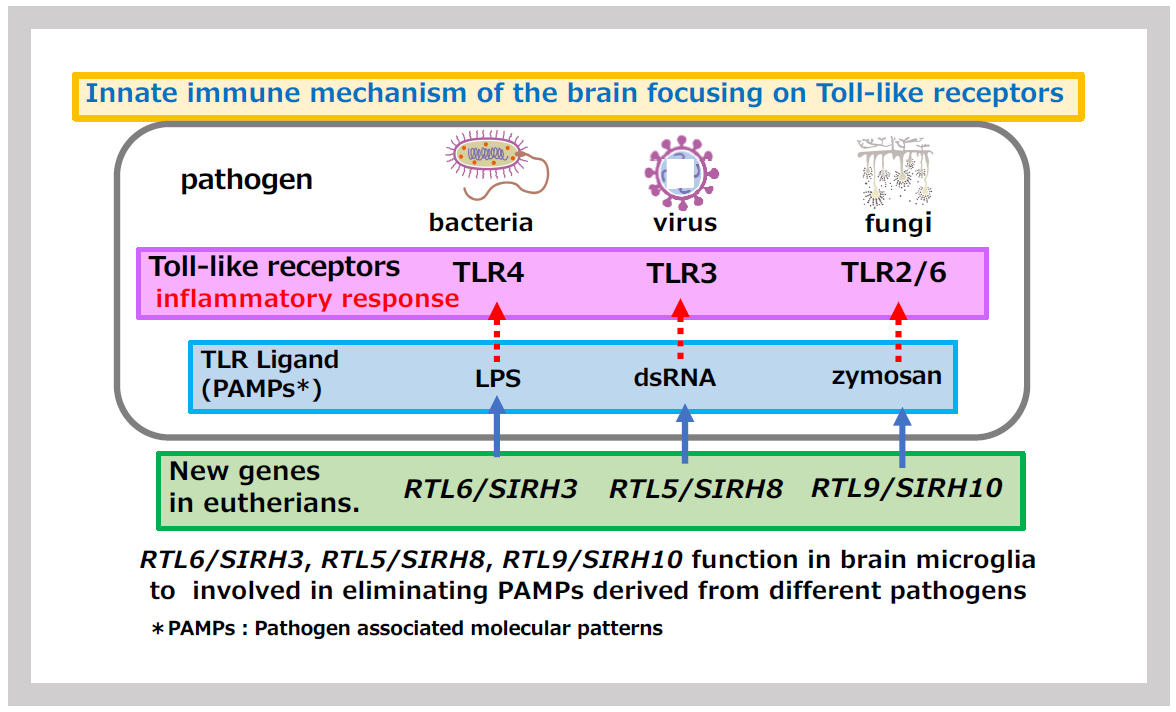
(1) SIRH3/RTL6 and SIRH8/RTL5
We first investigated the functions of SIRH3/RTL6 and SIRH8/RTL5 because SIRH3/RTL6 is particularly highly conserved among the SIRH/RTL genes, suggesting it plays a critical role in eutherians. Additionally, SIRH8/RTL5 is phylogenetically the closest to SIRH3/RTL61 (refer to (3)).
Using knock-in (KI) mice, where fluorescent proteins (Venus or mCherry) were fused to SIRH3/RTL6 and SIRH8/RTL5 proteins, we observed that these fusion proteins were produced in microglia and widely distributed throughout the brain as secreted proteins. Upon administration of LPS, SIRH3/RTL6 protein responded rapidly, forming a complex with LPS1 (Figures 27 and 28).
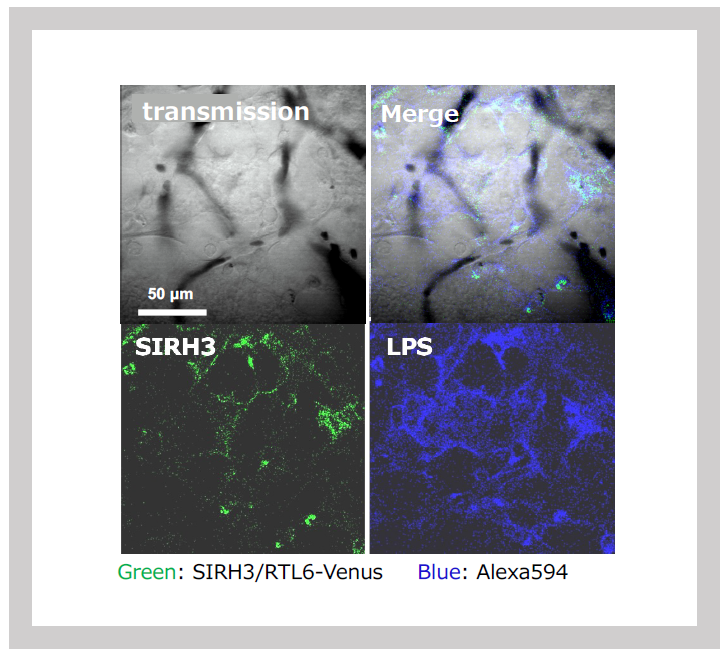
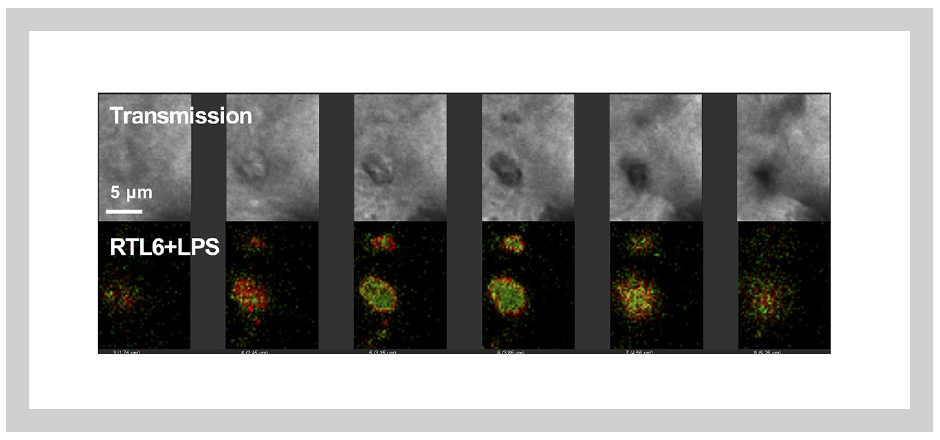
Green: SIRH3/RTL6-Venus, Red: LPS-Alexa594. Three-dimensional sectioning shows that the SIRH3/RTL6 protein (green) forms the central core of the complex, with LPS (red) trapped on its surface.
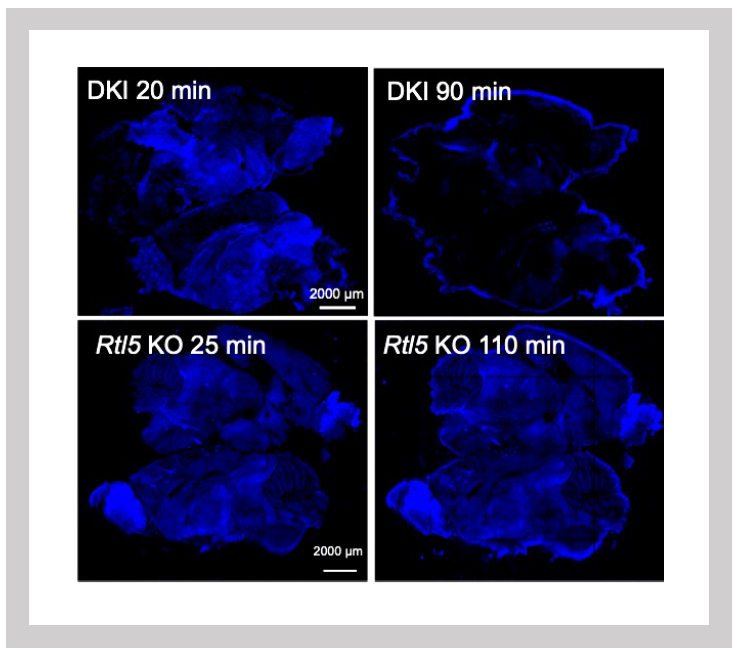
Degradation of dsRNA proceeds in double KI mice carrying the Sirh3-Venus and Sirh8-mCherry alleles, but persists in Sirh8/Rtl5 KO mice.
Blue: dsRNA-Rhodamine
On the other hand, the SIRH8/RTL5 protein primarily formed specific complexes with other virus-derived PAMPs, such as dsRNA and unmethylated DNA, facilitating their elimination. This response was absent in Sirh8/Rtl5 KO mice1 (Figure 29).
In other words, SIRH3/RTL6 and SIRH8/RTL5 proteins serve as frontline defenders of the brain's innate immune system, remaining on constant alert for pathogen invasion. When pathogens enter, these proteins respond swiftly to capture PAMPs. While the innate immune system is an evolutionarily conserved mechanism present in nearly all animals—and although microglia have long been known to process foreign substances—our findings reveal that it has undergone unique adaptations in eutherians, giving rise to specialized proteins that enhance the efficiency of this elimination process.
SIRH3/RTL6 and SIRH8/RTL5 are genes present only in eutherians, including humans. Originally derived from retroviruses, these genes have been repurposed to play a defensive role against pathogens—much like a captured piece in the Japanese game of shogi, redeployed to serve a new purpose (Note 1). This raises an intriguing question: Do other animals also possess similar genes or proteins that directly bind to PAMPs and promote their clearance?
Note 1: In Shogi (Japanese chess), captured opponent pieces can be reused as one's own when needed. This unique rule is a distinctive feature of Shogi.
- Irie M, Itoh J, Matsuzawa A, Ikawa M, Kiyonari H, Kihara M, Suzuki T, Hiraoka Y, Ishino F and Kaneko-Ishino T. Retrovirus-derived RTL5 and RTL6 genes are novel constituents of the innate immune system in the eutherian brain. Development 149: dev200976 (2022).
(2) SIRH10/RTL9
Next, we investigated SIRH10/RTL9. Unlike SIRH3/RTL6 and SIRH8/RTL5, the SIRH10/RTL9 protein is a significantly larger protein. Its N-terminal and central regions contain amino acid sequences unrelated to GAG, instead showing homology to the Herpes virus-derived outer envelope glycoprotein BLLF1 and the large tegument protein UL36. The GAG-derived portion is confined to the C-terminal one-third of the protein.
To examine its cellular localization, we generated knock-in (KI) mice expressing an mCherry-tagged SIRH10/RTL9 protein. Fluorescence imaging revealed that SIRH10/RTL9 localizes to microglial lysosomes2 (Figure 30). Zymosan, a component of fungal cell walls, is rapidly taken up by microglia and macrophages, transported to lysosomes and degraded. Upon administering zymosan to the brains of KI mice, we observed co-localization of SIRH10/RTL9 and zymosan within lysosomes2.
To further investigate the function of the GAG-derived portion in SIRH10/RTL9, we generated Sirh10/RTL9 KO mice lacking the C-terminal one-third of the protein, which contains the GAG-like region. In these KO mice, although zymosan was successfully taken up into lysosomes, its degradation was impaired (Figure 31). These findings suggest that SIRH10/RTL9 is crucial for the degradation of zymosan and thus plays a protective role against fungal infections in the brain2.
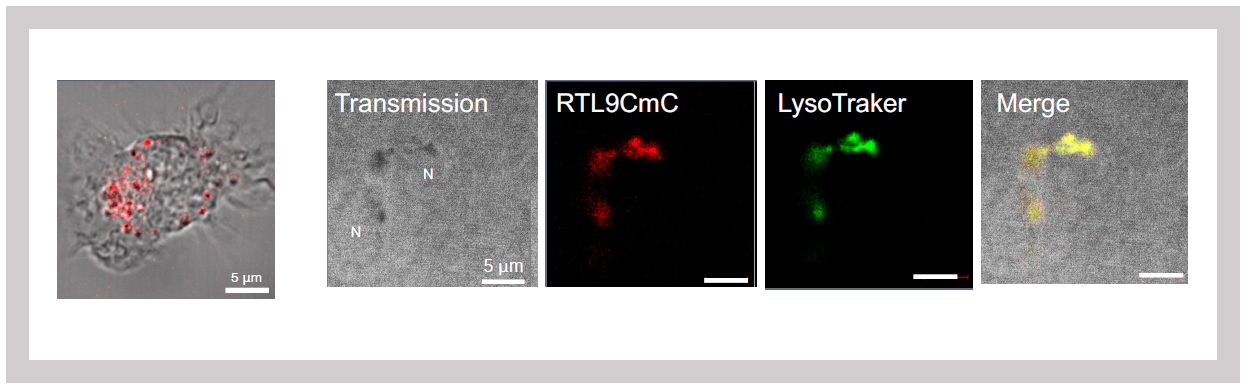
Left: SIRH10/RTL9 expression in isolated microglia
Right: SIRH10/RTL9 signal colocalizes with LysoTracker, a dye that specifically stains lysosomes.
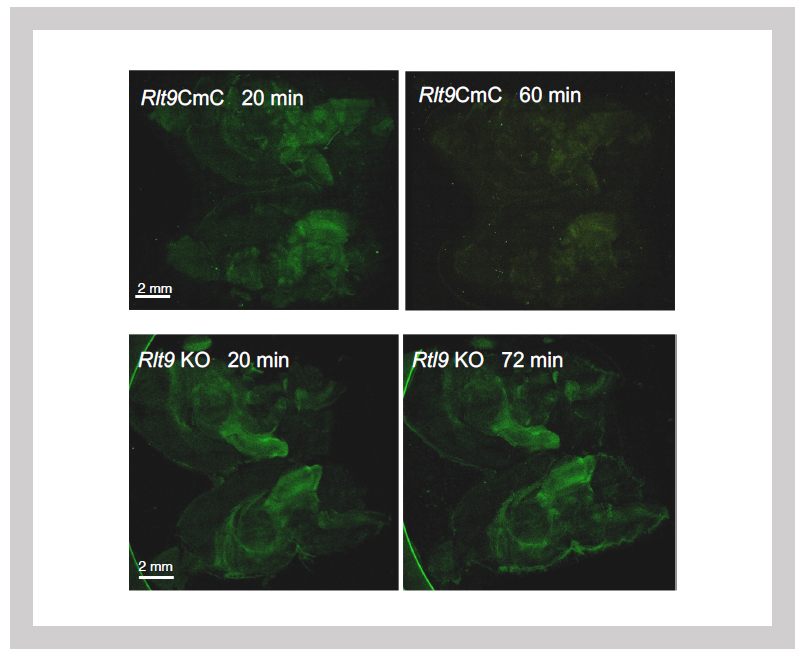
Green: Zymosan fluorescence signal
Top: Zymosan is processed at 60 minutes in Sirh10/Rtl9-mCherry knock-in mice.
Bottom: It remains unprocessed after 72 minutes in Sirh10/Rtl9 KO mice.
- Ishino F, Itoh J, Irie M, Matsuzawa A, Naruse M, Suzuki T, Hiraoka Y and Kaneko-Ishino T. Retrovirus-derived RTL9 plays an important role in innate antifungal immunity in the eutherian brain. Int J Mol Sci 24:14884 (2023).
(3) How Did the SIRH3/RTL6 Protein Originate?
SIRH3/RTL6 exhibits exceptionally high evolutionary conservation—surpassing even the average conservation of housekeeping genes involved in essential cellular functions (Table 1). This suggests that protecting the brain from bacterial infections has been a critical evolutionary priority in eutherians.
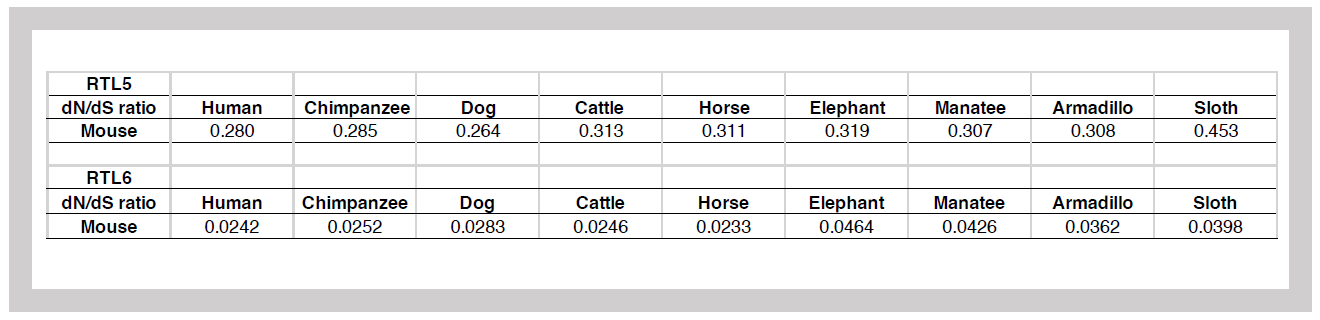
dN/dS is a value calculated by the ratio of synonymous (S) to non-synonymous (N) substitutions in the amino acids encoded by a gene. A ratio less than 1 indicates high evolutionary conservation. The average for housekeeping genes is approximately 0.093, and the gene with the highest conservation, Histone H3, has a dN/dS ratio of <0.01. This suggests that SIRH3/RTL6 is highly conserved and likely performs an essential function.
A key characteristic of SIRH3/RTL6 is its extremely basic isoelectric point (pI) of 11.15. Compared to the original GAG protein, SIRH3/RTL6 has undergone significant amino acid sequence changes, sharing only 27.7% identity (44.1% similarity). The N-terminal region contains a leucine zipper motif, while the C-terminal region features a cluster of basic amino acids (pI = 12.0), which contributes to the extreme basicity of the entire SIRH3 protein. This structural adaptation aligns well with its function—LPS, the binding target of SIRH3/RTL6, is an extremely acidic molecule with a pI of 2.0. In other words, SIRH3/RTL6 has evolved specifically to bind LPS efficiently, reinforcing its crucial role in bacterial defense.
Originally, viral GAG proteins are cleaved into three parts by the POL protease, forming the matrix, the capsid that houses the RNA genome, and the nucleocapsid that binds to the RNA genome. Specifically, the capsid-homologous portion of SIRH3/RTL6 exhibits self-assembling properties. The C-terminal region, which contains a basic helical structure, enables the protein to effectively capture LPS. Additionally, the N-terminal leucine zipper structure likely enhances the protein's ability to form dimers or multimers (Figure 32). When observed in complex form, SIRH3/RTL6 appeared as a core structure with LPS bound to the surface (Figure 28).
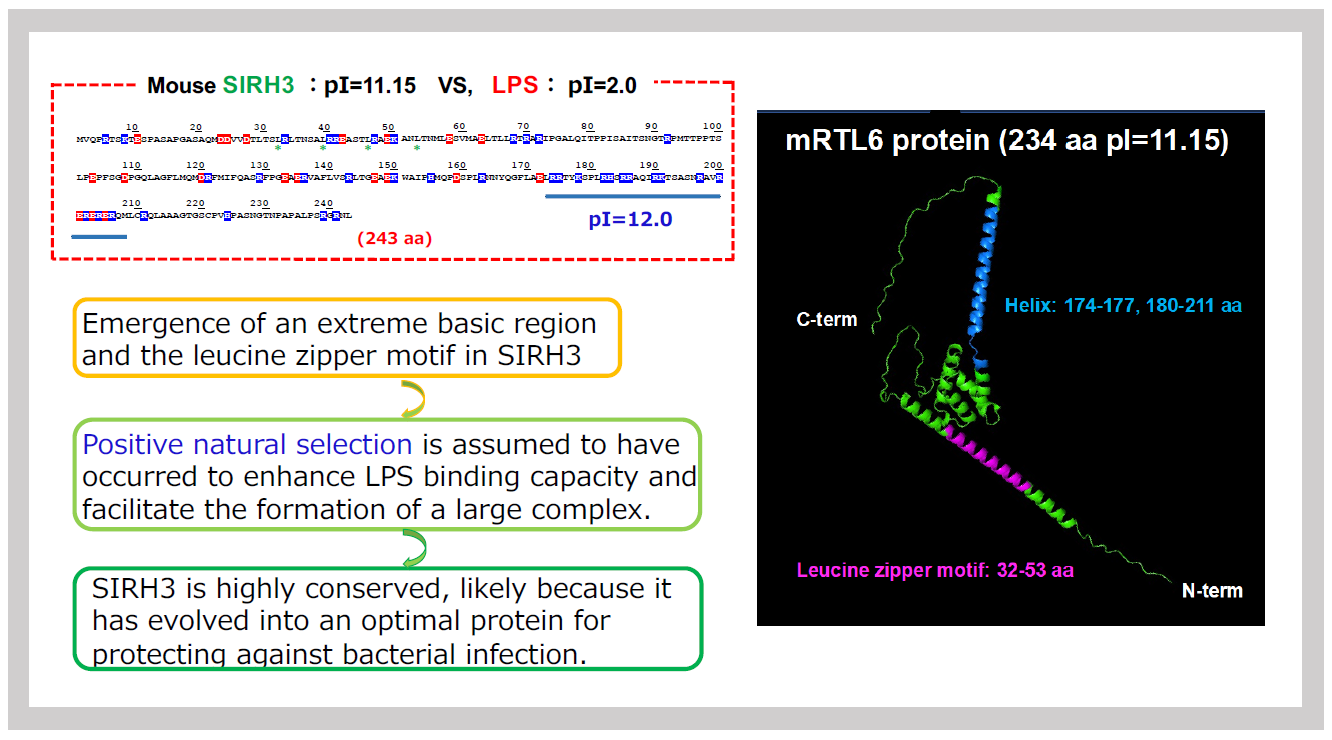
The amino acid sequence on the left indicates the position of the leucine zipper structure and the basic sequence-lined structure. The 3D protein structure of SIRH3, estimated by AlphaFold 2, is shown on the right.
These structural features indicate that SIRH3/RTL6 has evolved to efficiently bind LPS and facilitate its removal. The emergence of the leucine zipper structure and the shift toward a more basic amino acid sequence would have required a substantial number of mutations if driven solely by random accumulation. A long evolutionary timeframe was likely required for these virus-derived genes to evolve into novel genes capable of combating bacterial infections after integrating into the mammalian genome. This gradual refinement, shaped by natural selection, exemplifies Darwinian evolution, where strong selective pressures transformed the gene into its current specialized function.
9-2: Natural Immune Defenders of the Brain from Stress: SIRH11/RTL4
In 2015, we reported that Sirh11/Zcchc16 (Rtl4) KO mice exhibit increased impulsivity, impaired short-term memory, and decreased adaptability to novel environments3. These mice also showed a delayed recovery of noradrenaline (NA) in the cortex (Spin-off 5). Interestingly, the human ZCCHC16 gene has been linked to rare mutations in patients with autism spectrum disorder (ASD)4-6. Despite its apparent importance, Sirh11/Zcchc16 gene expression is extremely low in the brain, leaving many unresolved questions. These include when, where, and in which cells (neurons or glial cells) it is expressed during development, as well as how it responds to NA stimulation.
To address these critical questions, we developed a KI mouse model in which the SIRH11/RTL4 protein was fused to a fluorescent protein, Venus, allowing us to visualize its expression in the brain. We analyzed its distribution and response to noradrenaline (NA) stimulation7. Our findings revealed SIRH11/RTL4 signals, appearing as small dots, in the hypothalamus, midbrain, hippocampus, and amygdala starting around postnatal day 107. Signal intensity gradually increased through the second and third weeks, stabilizing at a slightly lower but consistent level. Additionally, SIRH11/RTL4 signals, approximately 1 μm in size, was also detected at the periphery of the thalamus, midbrain aqueduct, and hippocampus immediately after birth, although the overall signal intensity remained low7.
The signal intensity in the hypothalamus and midbrain responded sensitively to stimuli. Even the simple act of transporting the mice from the breeding room to the experimental room caused an increase in signal intensity. Furthermore, administering isoproterenol, a β-adrenergic receptor agonist, significantly enhanced the signal intensity7 (Figure 33). Conversely, the signal intensity decreased under isoflurane anesthesia. However, when milnacipran, a reuptake inhibitor for both noradrenaline (NA) and serotonin, was administered during anesthesia, the signal intensity remained stable7 (Figure 34).
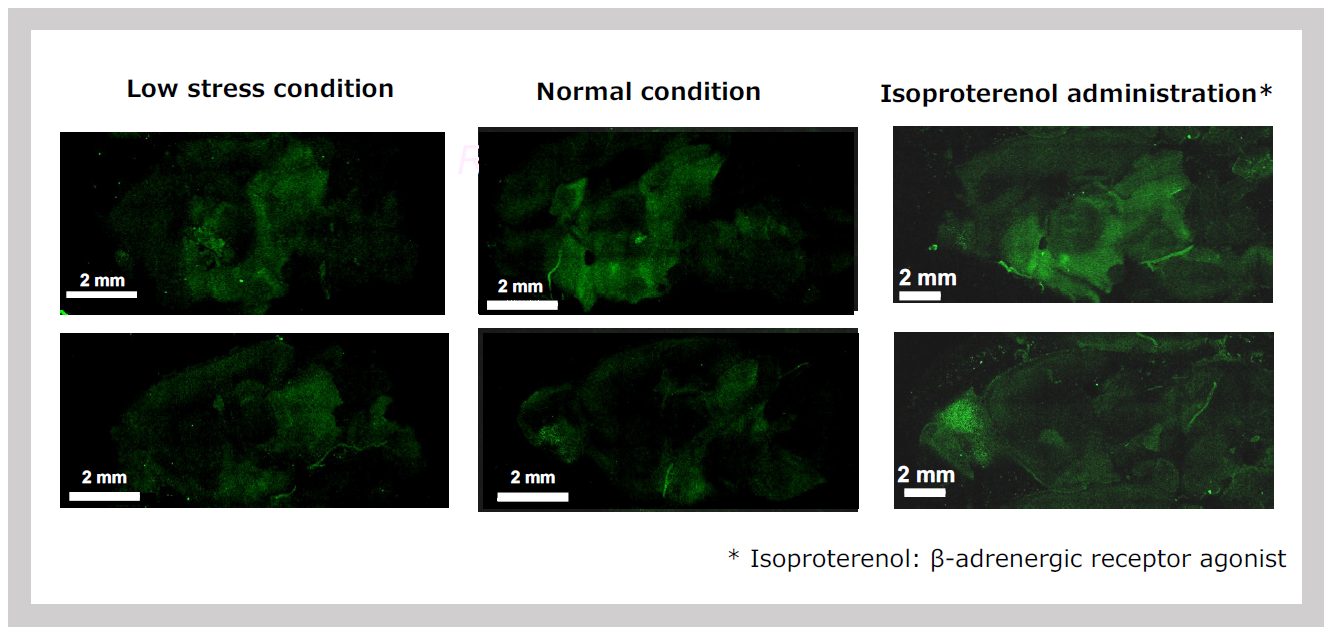
The SIRH11 signal is elevated simply by transporting the animals from the breeding room (left) to the laboratory (center) and is further enhanced by the administration of isoproterenol, which mimics the action of noradrenaline (NA).
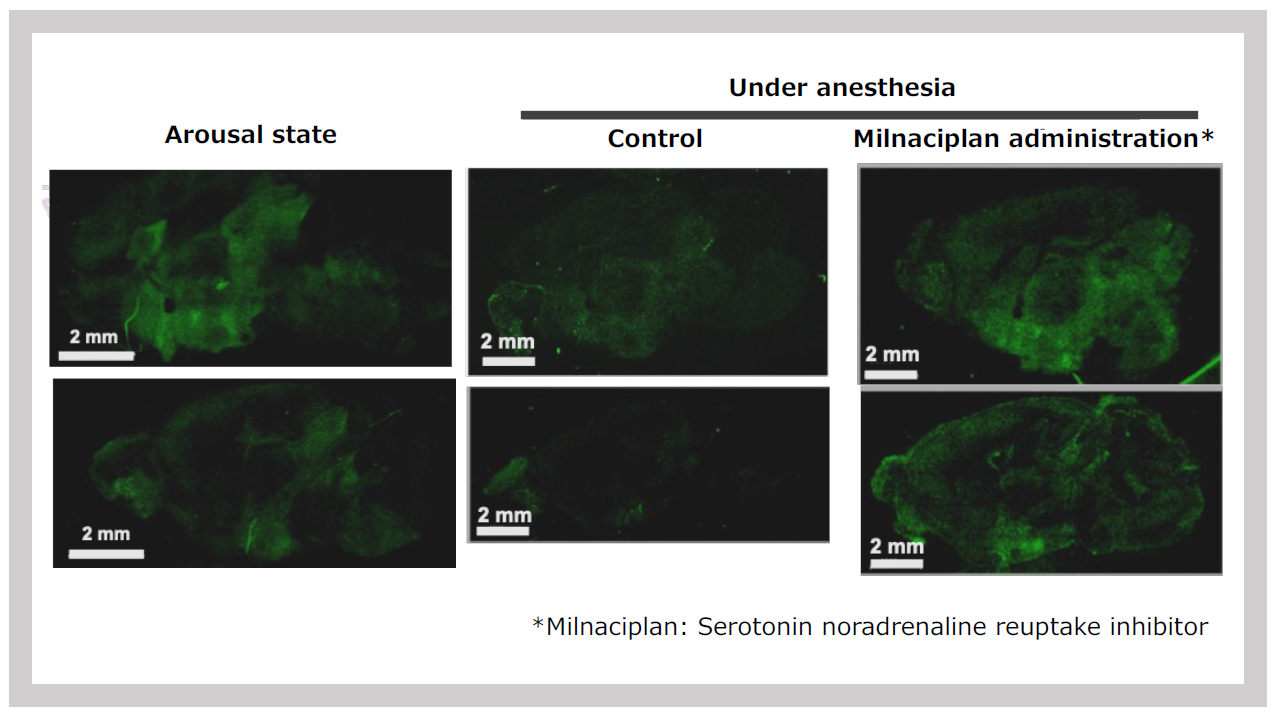
SIRH11 signal intensity (left) is significantly reduced under anesthesia (center), but the signal is maintained when milnacipran, a serotonin and noradrenaline reuptake inhibitor, is administered during anesthesia.
Since it exists as a secreted protein within the cell, we hypothesized that, like SIRH3/RTL6 and SIRH8/RTL5 proteins, it would be expressed in microglia. To test this, we examined co-cultures of microglia and astrocytes and observed a microglia-specific signal (Figure 35). Additionally, we captured the process of signal release from microglia into the medium following isoproterenol stimulation. These findings indicate that SIRH11/RTL4 protein is expressed in microglia and is released extracellularly in response to NA stimulation7.
Furthermore, fluorescence intensity in the brain increased in response to NA-related signals, such as stress, and was found to depend on the state of arousal. The presence of released NA stabilized the signal, indicating that this protein is involved in stress responses mediated by NA.
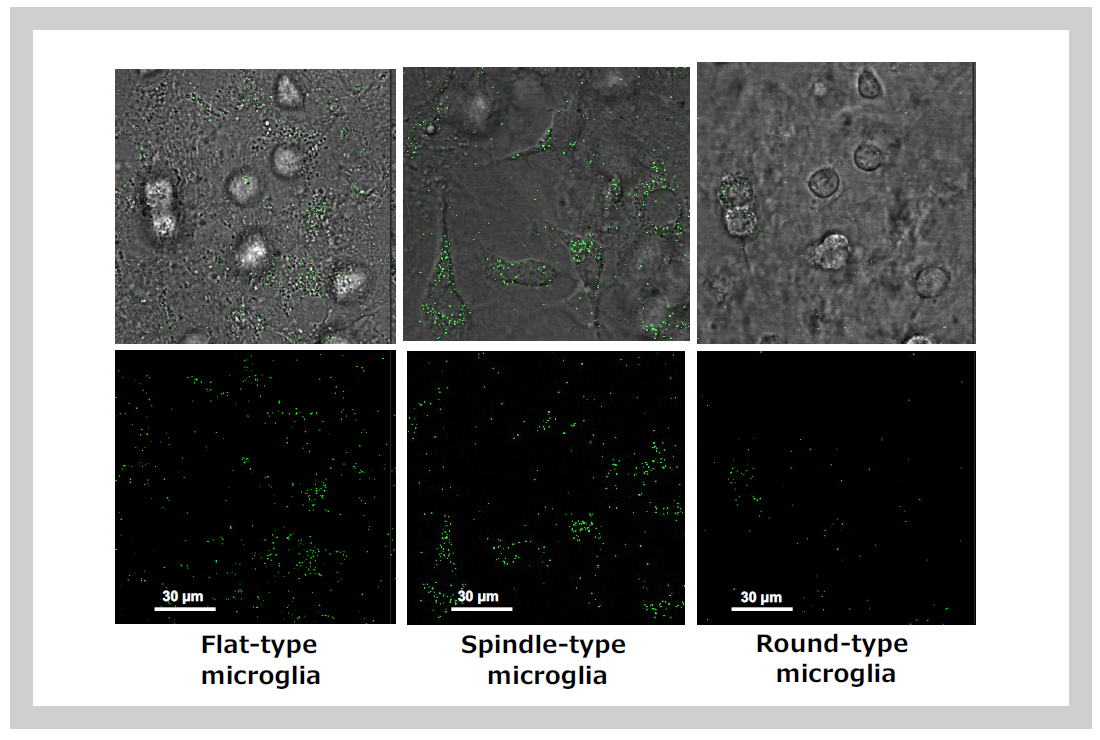
When mixed glial cultures are prepared from neonatal brains, SIRH11 signals are detected in various types of microglia: flat microglia growing within astrocyte feeder cells (left), spindle-shaped microglia on feeder cells (center), and round microglia floating in the medium (right).
The timing of SIRH11/RTL4 expression coincides with the development of the NA neural network, which is crucial for a newborn's adaptation to a new environment. This suggests that the secretion of this protein likely plays a vital role in the proper development of the brain's neural system in response to new stimuli.
In Sirh11/Zcchc16 (Rtl4) KO mice, this process is disrupted, potentially contributing to ASD-like abnormalities. Furthermore, it is well established that NA can induce apoptosis in various cells, including neurons. While NA is essential for survival, its toxicity raises the possibility that SIRH11/RTL4 functions to protect cells—such as neuron and microglia themselves—from this toxicity. It might also play a role in processing apoptotic cell fragments. Unlike the other SIRH/RTL genes mentioned earlier, which protect the brain from pathogen infections, SIRH11/RTL4 is believed to be involved in responding to psychological stress. The acquisition of this gene may have enabled eutherians to safely and efficiently utilize NA signaling, giving them an advantage in coping with stress7.
- Irie M, Yoshikawa M, Ono R, Iwafune H, Furuse T, Yamada I, Wakana S, Yamashita Y, Abe T, Ishino F and Kaneko-Ishino T. Cognitive function related to the Sirh11/Zcchc16 gene acquired from an LTR retrotransposon in eutherians. PLoS Genet 11, e1005521 (2015).
- Lim ET, Raychaudhuri S, Sanders SJ, Stevens C, Sabo A, MacArthur DG, Neale BM, Kirby A, Ruderfer DM, Fromer M et al. Rare complete knockouts in humans: Population distribution and significant role in autism spectrum disorders. Neuron 77, 235–242 (2013).
- Boles RG. (University of California School of Medicine, Irvine, CA, USA). Boy with autism and frameshift variant in ZCCHC16. Personal communication (2017).
- Fernández-Jaén, A. (Universidad Europea de Madrid, Madrid, Spain). ZCCHC16 mutation, intellectual disability and autism. Personal communication (2021).
- Ishino F, Itoh J, Matsuzawa A, Irie M, Suzuki To, Hiraoka Y, Yoshikawa M and Kaneko-Ishino T. RTL4, a retrovirus-derived gene implicated in autism spectrum disorder, is a microglial gene that responds to noradrenaline in the postnatal brain. Int J Mol Sci 26: 13738 (2024).
Theme 10: The placenta as a laboratory for evolution—The role of the placenta in further acquisition of virus-derived genes—
“The placenta”serves as a fascinating example of an innovative evolution, where virus-derived genes have been repurposed to perform vital functions in its development. Through the acquisition of virus-derived genes, the placenta has gained the ability to perform complex tasks necessary for fetal survival, such as regulating blood flow, supporting fetal growth, and facilitating gas exchange between the mother and the developing fetus. Moreover, we propose that once the placenta was established, it has likely served as a site for further gene acquisition from viruses, contributing to the advancement of mammalian evolution. In this sense, “the placenta can be considered an experimental laboratory for mammalian evolution”.
So far, we have introduced three acquired genes that play essential roles in the survival of the fetus and newborn: PEG10, which is involved in the early formation of the placenta1 (Theme 4); PEG11/RTL1, which maintains fetal capillaries in the placenta2 (Theme 5); and Sirh7/Ldoc1, which functions in the endocrine regulation of the placenta3 (Theme 5). Subsequent analyses have revealed that PEG10 also plays a vital role in maintaining fetal capillaries during gestation4.
PEG10 has virus-derived features, including a protease with a DSG motif. Our studies have shown that a mutation in this motif can disrupt placental function at the perinatal stage, leading to fetal death rather than early embryonic lethality (Figure 36). Examination of the placenta revealed that the structure of the fetal capillaries was severely disrupted, differing from the PEG11/RTL1 KO case.
Another virus-derived feature of PEG10 is the presence of ORF1 and ORF2, derived from GAG and POL, which produce two proteins: ORF1 alone and the fusion protein ORF1-ORF2. Mutants lacking ORF1-ORF2 exhibited early embryonic lethality, similar to Peg10 KO, while mutants lacking the ORF1 alone exhibited mid-gestation lethality (Shiura et al., in preparation).This defect is also associated with placental abnormalities, indicating that PEG10 is a multifunctional and essential placental gene, playing a critical role in placental function from the early stages of placental formation through to the mid and late stages of gestation. These evidences highlight the role of virus-derived genes in placental evolution, which has shaped mechanisms to support mammalian reproduction.
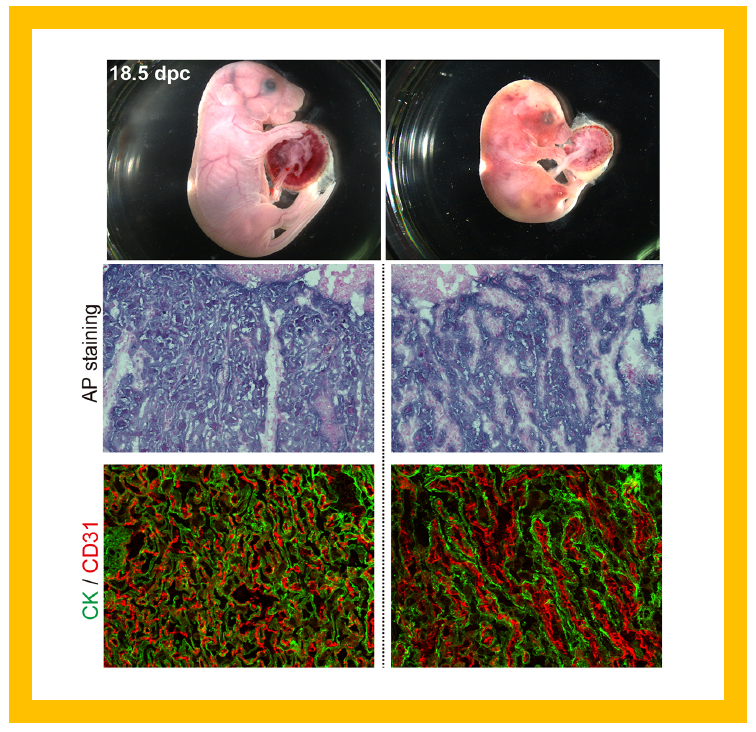
The mutant mice exhibit lethality during the perinatal period, immediately before and after birth (upper panel). The cause is a disruption in the labyrinthine layer of fetal capillaries in the placenta, where trophoblast cells, stained with alkaline phosphatase (AP staining: middle row) and cytokeratin (green in CK staining: lower row), have fused. The fetal capillary endothelial cells stained with CD31 antibody (red: lower row), inside the trophoblast layers have also fused, resulting in the destruction of the entire fetal capillary network.
Figure 37 summarizes the virus-derived acquired genes that perform essential functions in the placenta. Peg10 is expressed in all the trophoblast cells across the three placenta layers: giant trophoblast, spongiotrophoblast and labyrinth. In Peg10 KO mice, the spongiotrophoblast and labyrinth layers fail to form, resulting in early embryonic lethality. In contrast, the PEG10-ASG mutant, which cannot maintain fetal capillaries in the labyrinth layer, causes perinatal lethality.
On the other hand, Peg11/Rtl1 is expressed in the endothelial cells of fetal capillaries, and Peg11/Rtl1 KO mice exhibit endothelial cell destruction, leading to late fetal lethality.
Similar to Peg10, Sirh7/Ldoc1 is expressed in all trophoblast cells, but its loss results in structural abnormalities in the three-layered placental architecture. Functionally, it impairs the production of placenta hormones, including progesterone (P4), placental lactogens 1 and 2, and various prolactins. Consequently, the timing of delivery is delayed, and neonatal lethality occurs due to maternal nursing failure (see Theme 5 and Figure 13). Thus, it is evident that abnormalities in the expression sites of each gene in the placenta lead to lethality ranging from early embryonic to neonatal stages.
In addition, two syncytin genes (syncytin A and B) play essential roles in the fusion of the two inner layers of syncytiotrophoblast cells surrounding the fetal capillaries in the placenta5, 6. The cell-fusion ability of Syncytin proteins originates from that of ENV proteins, which were originally necessary for viral to fuse with host cells. Unlike the SIRH/RTL genes, which are conserved in eutherians, syncytin genes were independently acquired from different viruses in each eutherian lineage. In humans, two distinct syncytin genes (syncytin 1 and 2) function in human placenta7,8.
The fact that at least five virus-derived acquired genes perform indispensable functions in the placenta strongly suggests that the acquisition of new genes from viruses played a crucial role in the emergence of the placenta as a novel organ in eutherians.
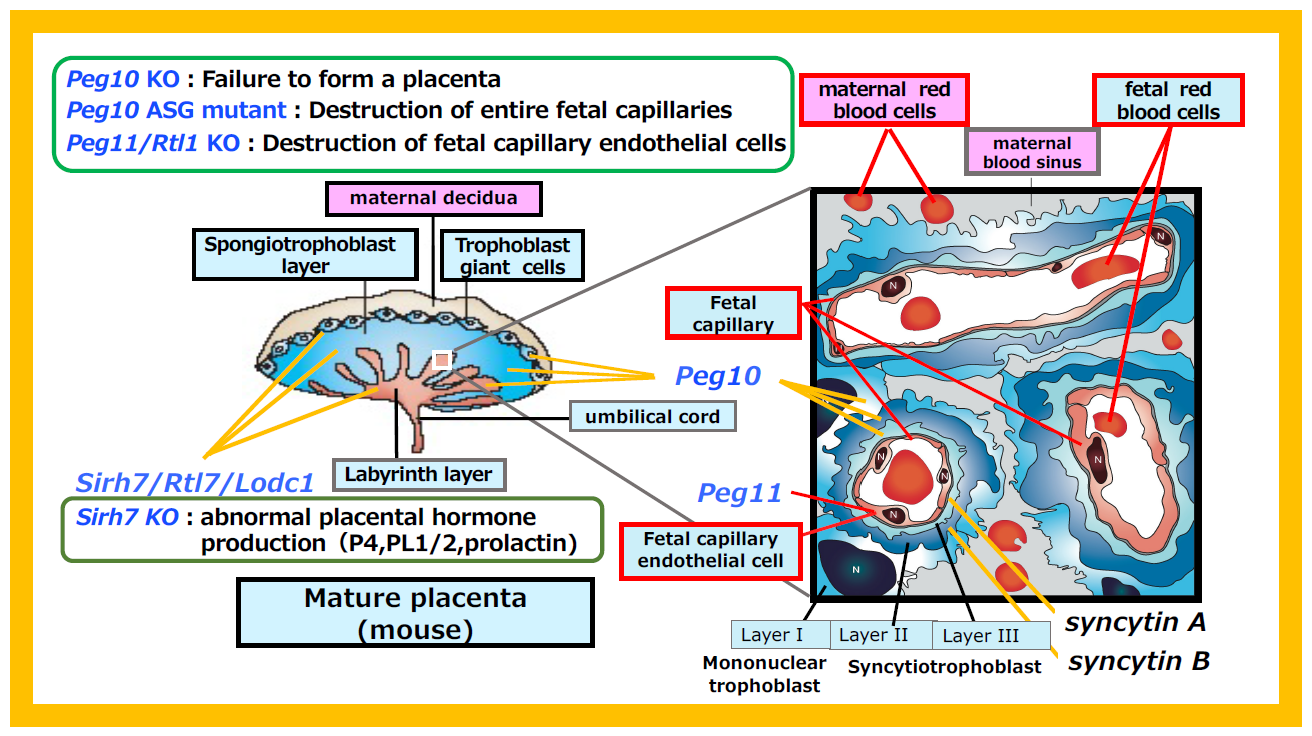
Supplement: Viral characteristics retained in PEG10
Retroviruses encode three proteins: GAG, POL, and ENV (see Spin-off 4).
- GAG is cleaved by the protease included in POL to produce three structural proteins essential for viral particle formation: the matrix that forms the outer layer; the capsid that encapsulates the entire RNA genome; and the nucleocapsid that aids in packaging the RNA genome. In this way, GAG contains all the proteins necessary to construct the viral structure.
- POL contains not only the aforementioned protease but also reverse transcriptase (RT), RNase H and DNA integrase (IN). These enzymes are individually processed by the protease, enabling the formation of double-stranded DNA from the viral RNA genome and the insertion of viral DNA into the host genome. POL thus contains all the enzymes required for these processes.
- ENV contains the enzymatic activity necessary for the virus to fuse with the host cell membrane.
Three major characteristics of retroviruses are retained in PEG10:
- PEG10 has two open reading frames (ORF1 and ORF2) derived from GAG and POL, respectively. ORF1 can be read independently, but through a "-1 frameshift mechanism," ORF1-ORF2 can also be read as a single protein, resulting in the production of two types of proteins from PEG10.
- The C-terminal of ORF1, corresponding to GAG, contains an RNA-binding domain (CCHC).
- At the beginning of ORF2, corresponding to POL, there is a protease domain (DSG).
Additionally, due to the protease activity derived from POL, these proteins are further cleaved into several fragments, each potentially having distinct functions.
Here, we would like to discuss two types of "virus-derived genes": "acquired genes" and "captured genes." Both SIRH/RTL genes—including PEG10, PEG11/RTL1 and SIRH3/LDOC1—and syncytin genes are "virus-derived genes," however, the terms "acquired gene" and "captured gene" are used differently to reflect their distinct acquisition processes.
Syncytin proteins are utilized for the fusion of syncytiotrophoblast cells in the placenta, retaining amino acid sequences almost unchanged from viral ENV proteins. Consequently, when the function of the newly integrated viral ENV gene became beneficial for a given lineage, the original gene was likely replaced, effectively transferring the functional role to the newly incorporated gene9,10. This process can be regarded as the capture of genes from retroviruses.
On the other hand, in the case of SIRH/RTL genes derived from GAG and POL genes, mutations have reduced their amino acid sequence similarity to 20–30%, transforming them into entirely new genes. As explained with the SIRH3/RTL6 protein (Theme 9, Figure 32), they have acquired novel functions while retaining the self-assembly property of GAG proteins. Similarly, PEG10 and PEG11/RTL1 have gained new functions through mutations, converting from their original viral GAG and POL to become essential proteins that support mammalian viviparity (Theme 5, 7, 10). In other words, “acquired genes “are those that have been extensively remodeled over long periods to become advantageous new traits for the host organism.
A group of SIRH/RTL genes, including SIRH3/RTL6, SIRH8/RTL5, SIRH10/RTL9 and SIRH11/RTL4, are expressed in microglia and function in the brain’s innate immunity. How do these genes relate to the group that functions in the placenta and how were they acquired from viruses? Unlike typical immune cells that originate from the bone marrow, microglia arise in the yolk sac during early development—before the formation of bone marrow—and migrate to the fetal brain, where they function throughout the organism’s life11. Notably, the yolk sac, like the placenta, is an extraembryonic tissue (Figure 38), suggesting the possibility that extraembryonic tissues serve as sites for gene acquisition.
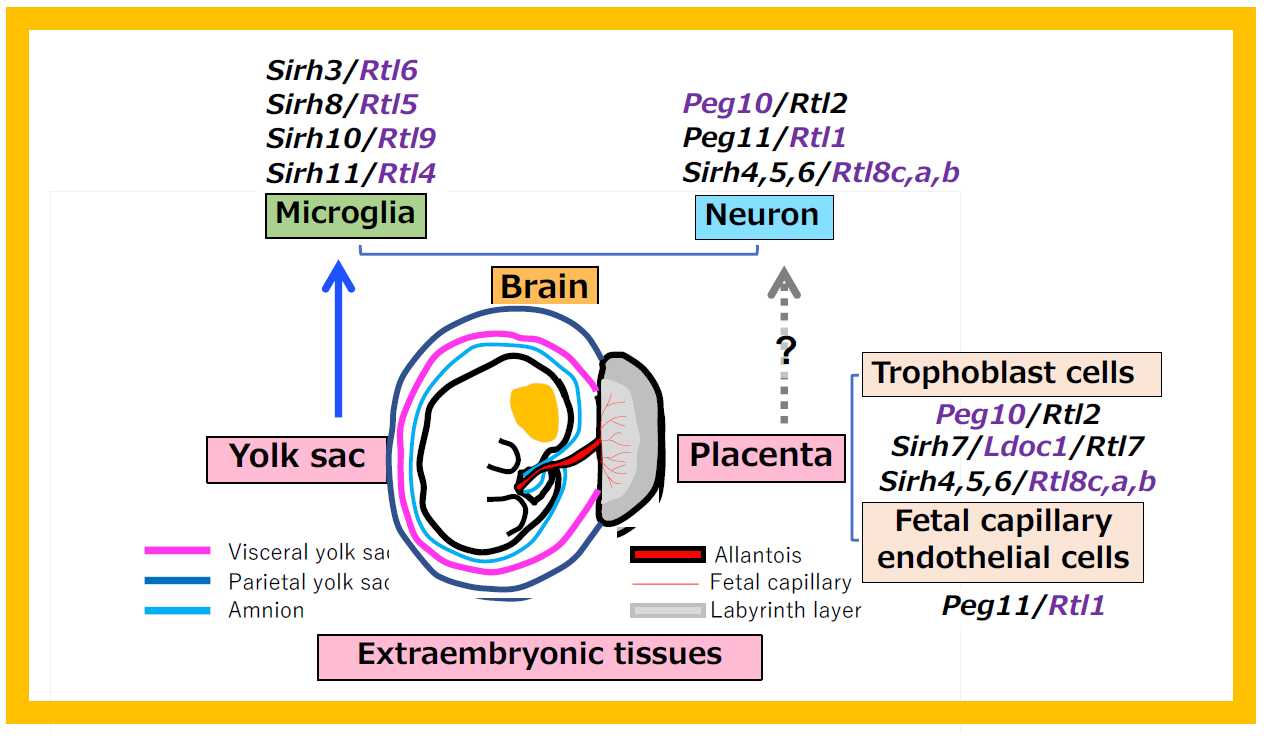
Endogenous retroviruses (ERVs) and transposons are completely suppressed in embryos (fetuses) due to high levels of DNA methylation. In contrast, extraembryonic tissues exhibit lower DNA methylation, allowing these genes to be expressed at low levels (leaky expression). It is conceivable that PEG10 was first acquired in the allantois—an extraembryonic tissue that serves as the precursor to the placenta—leading to the emergence of the placenta. Subsequently, the placenta may have acted as a platform for further gene acquisition from viruses, accelerating mammalian evolution. In this sense, the placenta(and yolk sac)can be regarded as an “experimental laboratory for mammalian evolution.”
The SIRH/RTL gene group consists of 11 genes in eutherians, but only two genes, PEG10 and SIRH12, are present in marsupials that possess a yolk sac placenta12. Additionally, another virus-derived gene group, the Paraneoplastic Ma-antigen (PNMA) gene family, is found in 12–15 genes in eutherians but only a single gene in marsupials13. This indicates that eutherians have a higher probability of acquiring genes compared to marsupials.
PEG10, PEG11/RTL1 and other SIRH/RTL genes are formally classified as LTR retrotransposon-derived GAG genes. The Sushi-ichi retrotransposon, with which they share higher homology, is an LTR retrotransposon now classified as part of the retrovirus-like LTR retrotransposon family Metaviridae. However, it is highly likely that these genes originated from three independent infection events of extinct retroviruses similar to the Sushi-ichi retrotransposon14, as they emerged suddenly in therian and eutherian mammals, and no Sushi-ichi retrotransposons are found in current mammalian lineages.
In vertebrates as a whole, endogenous retroviruses are widely distributed across species (in humans, they make up about 9% of the genome). However, the likelihood of these elements being incorporated into functional genes is very low, with the vast majority of such cases occurring within the mammalian lineage15. Considering all animals and plants, gene acquisition from LTR retrotransposons, as well as from retroviruses, is also rare. However, it is notably concentrated in mammals and angiosperms16. These data support our hypothesis that "the placenta is an experimental laboratory for mammalian evolution."
Angiosperms, similar to mammals with viviparity, produce seeds within the cotyledon (the lower part of the pistil) and also possess a genomic imprinting mechanism17. It is intriguing to consider whether the frequency of novel gene acquisition events is linked to the presence of genomic imprinting mechanisms—an area that warrants further investigation in future research.
- Ono R, Nakamura K, Inoue K, Naruse M, Usami T, Wakisaka-Saito N, Hino T, Suzuki-Migishima R, Ogonuki N, Miki H, Kohda T, Ogura A, Yokoyama M, Kaneko-Ishino T, and Ishino F. Deletion of Peg10, an imprinted gene acquired from a retrotransposon, causes early embryonic lethality. Nat Genet 38, 101–106 (2006).
- Sekita Y, Wagatsuma H, Nakamura K, Ono R, Kagami M, Wakisaka-Saito N, Hino T, Suzuki-Migishima R, Kohda T, Ogura A, Ogata T, Yokoyama M, Kaneko-Ishino T* and Ishino F*. Role of retrotransposon-derived imprinted gene, Rtl1, in the feto-maternal interface of mouse placenta. Nat Genet 40, 243–248 (2008).
- Naruse M, Ono R, Irie M, Nakamura K, Furuse T, Hino T, Oda K, Kashimura M, Yamada I, Wakana S, Yokoyama M, Ishino F, Kaneko-Ishino T. Sirh7/Ldoc1 knockout mice exhibit placental P4 overproduction and delayed parturition. Development 141, 4763–4771 (2014).
- Shiura H, Ono R, Tachibana S, Kohda T, Kaneko-Ishino T* and Ishino F*. PEG10 viral aspartic protease domain is essential for the maintenance of fetal capillary structure in the mouse placenta. Development 148:dev199564 (2021).
- Dupressoir A, Vernochet C, Bawa O, Harper F, Pierron G, Opolon P, Heidmann T. Syncytin-A knockout mice demonstrate the critical role in placentation of a fusogenic, endogenous retrovirus-derived, envelope gene. Proc Natl Acad Sci U S A. 106,12127–12132 (2009).
- Dupressoir A, Vernochet C, Harper F, Guégan J, Dessen P, Pierron G, Heidmann T. A pair of co-opted retroviral envelope syncytin genes is required for formation of the two-layered murine placental syncytiotrophoblast. Proc Natl Acad Sci U S A. 108, E1164–1173 (2011).
- Mi S, Lee X, Li X, Veldman GM, Finnerty H, Racie L, LaVallie E, Tang XY, Edouard P, Howes S, Keith JC Jr, McCoy JM. Syncytin is a captive retroviral envelope protein involved in human placental morphogenesis. Nature 403, 785–789 (2000).
- Blaise S, de Parseval N, Bénit L, Heidmann T. Genomewide screening for fusogenic human endogenous retrovirus envelopes identifies syncytin 2, a gene conserved on primate evolution. Proc Natl Acad Sci U S A 100, 13013–13018 (2003).
- Lavialle C, Cornelis G, Dupressoir A, Esnault C, Heidmann O, Vernochet C, Heidmann T. Paleovirology of 'syncytins', retroviral env genes exapted for a role in placentation. Philos Trans R Soc Lond B Biol Sci 368:20120507 (2013).
- Imakawa K, Nakagawa S, Miyazawa T. Baton pass hypothesis: successive incorporation of unconserved endogenous retroviral genes for placentation during mammalian evolution. Genes Cells 20, 771–788 (2015).
- Ginhoux F, Greter M, Leboeuf M, Nandi S, See P, Gokhan S, Mehler MF, Conway SJ, Ng LG, Stanley ER, Samokhvalov IM, Merad M. Fate mapping analysis reveals that adult microglia derive from primitive macrophages. Science 330, 841–845 (2010).
- Ono R, Kuroki Y, Naruse M, Ishii M, Iwasaki S, Toyoda A, Fujiyama A, Shaw G, Renfree M B, Kaneko-Ishino T and Ishino F. Identification of SIRH12, a retrotransposon-derived gene specific to marsupial mammals. DNA Res 18, 211–219 (2011).
- Iwasaki S, Suzuki S, Clark H, Ono R, Shaw G, Renfree M B, Kaneko-Ishino T and Ishino F. Identification of novel PNMA-MS1 in marsupials suggests LTR retrotransposon-derived PNMA genes differently expanded in marsupials and eutherians. DNA Res 20, 425–436 (2013).
- Henriques WS, Young JM, Nemudryi A, Nemudraia A, Wiedenheft B, Malik HS. The Diverse Evolutionary Histories of Domesticated Metaviral Capsid Genes in Mammals. Mol Biol Evol 41: msae061 (2024).
- Wang J, Han GZ. Frequent Retroviral Gene Co-option during the Evolution of Vertebrates. Mol Biol Evol 37, 3232–3242 (2020).
- Wang J, Han GZ. Unearthing LTR Retrotransposon gag Genes Co-opted in the Deep Evolution of Eukaryotes. Mol Biol Evol 38, 3267–3278 (2021).
- Köhler C, Weinhofer-Molisch I. Mechanisms and evolution of genomic imprinting in plants. Heredity105, 57–63 (2010).